A major proposed upgrade of the LHCb detector for LHC Runs 5 and 6 would allow a wide range of flavour-physics observables to be explored with extreme precision. Lucia Grillo, Stefano Perazzini and Dorothea vom Bruch offer a snapshot of LHCb Upgrade II status and plans.
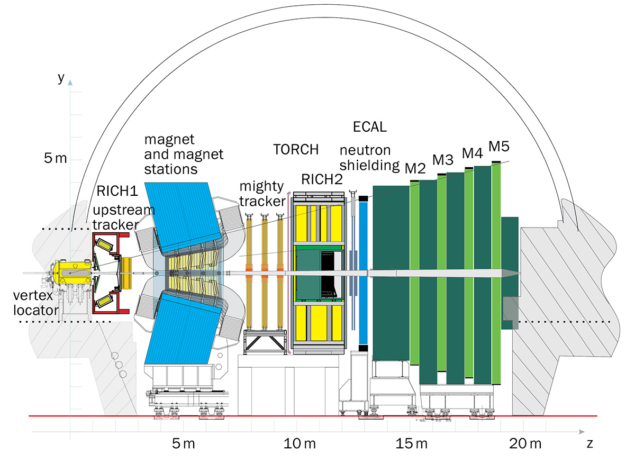
The LHCb collaboration is never idle. While building and commissioning its brand new Upgrade I detector, which entered operation last year with the start of LHC Run 3, planning for Upgrade II was already under way. This proposed new detector, envisioned to be installed during Long Shutdown 4 in time for High-Luminosity LHC (HL-LHC) operations continuing in Run 5, scheduled to begin in 2034/2035, would operate at a peak luminosity of 1.5 × 1034 cm–2 s–1. This is 7.5 times higher than at Run 3 and would generate data samples of heavy-flavoured hadron decays six times larger than those obtainable at the LHC, allowing the collaboration to explore a wide range of flavour-physics observables with extreme precision. Unprecedented tests of the CP-violation paradigm (see “On point” figure) and searches for new physics at double the mass scales possible during Run 3 are among the physics goals on offer.
Attaining the same excellent performance as the original detector has been a pivotal constraint in the design of LHCb Upgrade I. While achieving the same in the much harsher collision environments at the HL-LHC remains the guiding principle for Upgrade II, the LHCb collaboration is investigating the possibilities to go even further. And these challenges need to be met while keeping the existing footprint and arrangement of the detector (see “Looking forward” figure). Radiation-hard and fast 3D silicon pixels, a new generation of extremely fast and efficient photodetectors, and front-end electronics chips based on 28 nm semiconductor technology are just a few examples of the innovations foreseen for LHCb Upgrade II, and will also set the direction of R&D for future experiments.
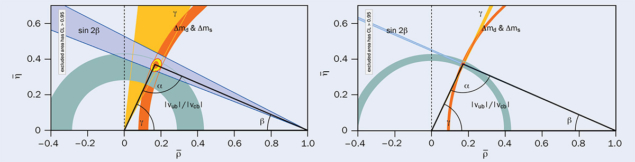
Rethinking the data acquisition, trigger and data processing, along with intense use of hardware accelerators such as field-programmable gate arrays (FPGAs) and graphics processing units (GPUs), will be fundamental to manage the expected five-times higher average data rate than in Upgrade I. The Upgrade II “framework technical design report”, completed in 2022, is also the first to consider the experiment’s energy consumption and greenhouse-gas emissions, as part of a close collaboration with CERN to define an effective environmental protection strategy.
Extreme tracking
At the maximum expected luminosity of the HL-LHC, around 2000 charged particles will be produced per bunch crossing within the LHCb apparatus. Efficiently reconstructing these particles and their associated decay vertices in real time represents a significant challenge. It requires the existing detector components to be modified to increase the granularity, reduce the amount of material and benefit from the use of precision timing.
The future VELO will be a true 4D-tracking detector
The new Vertex Locator (VELO) will be based, as it was for Upgrade I (CERN Courier May/June 2022 p38), on high-granularity pixels operated in vacuum in close proximity to the LHC beams. For Upgrade II, the trigger and online reconstruction will rely on the selection of events, or parts of events, with displaced tracks at the early stage of the event. The VELO must therefore be capable of independently reconstructing primary vertices and identifying displaced tracks, while coping with a dramatic increase in event rate and radiation dose. Excellent spatial resolution will not be sufficient, given the large density of primary interactions along the beam axis expected under HL-LHC conditions. A new coordinate – time – must be introduced. The future VELO will be a true 4D-tracking detector that includes timing information with a precision of better than 50 ps per hit, leading to a track time-stamp resolution of about 20 ps (see “Precision timing” figure).
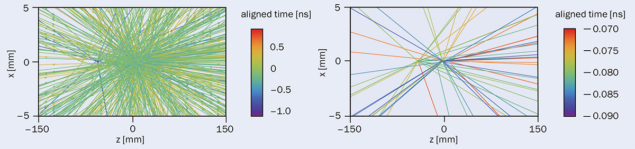
The new VELO sensors, which include 28 nm technology application-specific integrated circuits (ASICs), will need to achieve this time resolution while being radiation-hard. The important goal of a 10 ps time resolution has recently been achieved with irradiated prototype 3D-trench silicon sensors. Depending on the rate-capability of the new detectors, the pitch may have to be reduced and the material budget significantly decreased to reach comparable spatial resolution to the current Run 3 detector. The VELO mechanics have to be redesigned, in particular to reduce the material of the radio-frequency foil that separates the secondary vacuum – where the sensors are located – from the machine vacuum. The detector must be built with micron-level precision to control systematic uncertainties.
The tracking system will take advantage of a detector located upstream of the dipole magnet, the Upstream Tracker (UT), and of a detector made of three tracking stations, the Mighty Tracker (MT), located downstream of the magnet. In conjunction with the VELO, the tracking system ensures the ability to reconstruct the trajectory of charged particles bending through the detector due to the magnetic field, and provides a high-precision momentum measurement for each particle. The track direction is a necessary input to the photon-ring searches in Ring Imaging Cherenkov (RICH) detectors, which identify the particle species. Efficient real-time charged-particle reconstruction in a very high particle-density environment requires not only good detector efficiency and granularity, but also the ability to quickly reject combinations of hits not produced by the same particle.
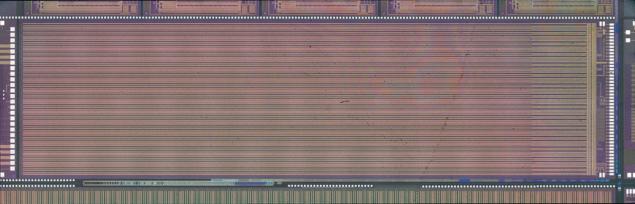
The UT and the inner region of the MT will be instrumented with high-granularity silicon pixels. The emerging radiation-hard monolithic active pixel sensor (MAPS) technology is a strong candidate for these detectors. LHCb Upgrade II would represent the first large-scale implementation of MAPS in a high-radiation environment, with the first prototypes currently being tested (see “Mighty pixels” figure). The outer region of the MT will be covered by scintillating fibres, as in Run 3, with significant developments foreseen to cope with the radiation damage. The availability of high-precision vertical-coordinate hit information in the tracking, provided for the first time in LHCb by pixels in the high-occupancy regions of the tracker, will be crucial to reject combinations of track segments or hits not produced by the same particle. To substantially extend the coverage of the tracking system to lower momenta, with consequent gains for physics measurements, the internal surfaces of the magnet side walls will be instrumented with scintillating bar detectors, the so-called magnet stations (MS).
Extreme particle identification
A key factor in the success of the LHCb experiment has been its excellent particle identification (PID) capabilities. PID is crucial to distinguish different decays with final-state topologies that are backgrounds to each other, and to tag the flavour of beauty mesons at production, which is a vital ingredient to many mixing and CP-violation measurements. For particle momenta from a few GeV/c up to 100 GeV/c, efficient hadron identification at LHCb is provided by two RICH detectors. Cherenkov light emitted by particles traversing the gaseous radiators of the RICHes is projected by mirrors onto a plane of photodetectors. To maintain Upgrade I performances, the maximum occupancy over the photodetector plane must be kept below 30%, the single-photon Cherenkov-angle resolution must be below 0.5 mrad, and the time resolution on single-photon hits should be well below 100 ps (see “RICH rewards” figure).
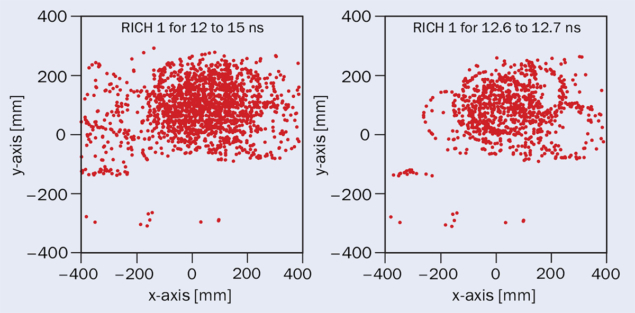
Next-generation silicon photomultipliers (SiPMs) with improved timing and a pixel size of 1 × 1 mm2, together with re-optimised optics, are deemed capable of delivering these specifications. The high “dark” rates of SiPMs, especially after elevated radiation doses, would be controlled with cryogenic cooling and neutron shielding. Vacuum tubes based on micro-channel plates (MCPs) are a potential alternative due to their excellent time resolution (30 ps) for single-photon hits and lower dark rate, but suffer in high-rate environments. New eco-friendly gaseous radiators with a lower refractive index can improve the PID performance at higher momenta (above 80 GeV/c), but meta-materials such as photonic crystals are also being studied. In the momentum region below 10 GeV/c, PID will profit from TORCH – an innovative 30 m2 time-of-flight detector consisting of quartz plates where charged particles produce Cherenkov light. The light propagates by internal reflection to arrays of high-granularity MCP–PMTs optimised to operate at high rates, with a prototype already showing performances close to the target of 70 ps per photon.
Excellent photon and π0 reconstruction and e–π separation are provided by LHCb’s electromagnetic calorimeter (ECAL). But the harsh occupancy conditions of the HL-LHC impose the development of 5D calorimetry, which complements precise position and energy measurements of electromagnetic clusters with a time resolution of about 20 ps. The most crowded inner regions will be equipped with so-called spaghetti calorimeter (SPACAL) technology, which consists of arrays of scintillating fibres either made of plastic or garnet crystals arranged along the beam direction, embedded in a lead or tungsten matrix. The less-crowded outer regions of the calorimeter will continue to be instrumented with the current “Shashlik” technology with refurbished modules and increased granularity. A timing layer, either based on MCPs or on alternated tungsten and silicon-sensor layers placed within the front and back ECAL sections, is also a possibility to achieve the ultimate time resolution. Several SPACAL prototypes have already demonstrated that time resolutions down to an impressive 15 ps are feasible (see “Spaghetti calorimetry” image).
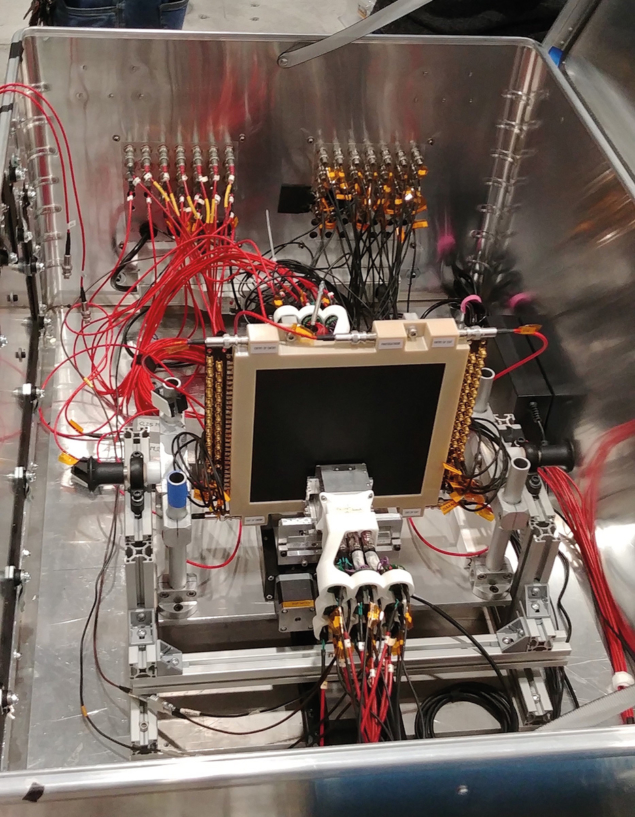
The final main LHCb subdetector is the muon system, based on four stations of multiwire proportional chambers (MWPCs) interleaved with iron absorbers. For Upgrade II, it is proposed that MWPCs in the inner regions, where the rate will be as high as a few MHz/cm2, are replaced with new-generation micro-pattern gaseous detectors, the micro-RWELL, a prototype of which has proved able to reach a detection efficiency of approximately 97% and a rate-capability of around 10 MHz/cm2. The outer regions, characterised by lower rates, will be instrumented either by reusing a large fraction (∼95%) of the current MWPCs or by implementing other solutions based on resistive plate chambers or scintillating-tile-based detectors. As with all Upgrade II subdetectors, dedicated ASICs in the front-end electronics, which integrate fast time-to-digital converters or high-frequency waveform samplers, will be necessary to measure time with the required precision.
Trigger and computing
The detectors for LHCb Upgrade II will produce data at a rate of up to 200 Tbit/s (see “On the up” figure), which for practical reasons needs to be reduced by four orders of magnitude before being written to permanent storage. The data acquisition therefore needs to be reliable, scalable and cost-efficient. It will consist of a single type of custom-made readout board combined with readily available data-centre hardware. The readout boards collect the data from the various sub-detectors using the radiation-hard, low-power GBit transceiver links developed at CERN and transfer the data to a farm of readout servers via next- generation “PCI Express” connections or Ethernet. For every collision, the information from the subdetectors is merged by passing through a local area network to the builder server farm.
With up to 40 proton–proton interactions, every bunch crossing at the HL-LHC will contain multiple heavy-flavour hadrons within the LHCb acceptance. For efficient event selection, hits not associated with the proton–proton collision of interest need to be discarded as early as possible in the data-processing chain. The real-time analysis system performs reconstruction and data reduction in two high-level-trigger (HLT) stages. HLT1 performs track reconstruction and partial PID to apply inclusive selections, after which the data is stored in a large disk buffer while alignment and calibration tasks run in semi real-time. The final data reduction occurs at the HLT2 level, with exclusive selections based on full offline-quality event reconstruction. Starting from Upgrade I, all HLT1 algorithms are running on a farm of GPUs, which enabled, for the first time at the LHC, track reconstruction to be performed at a rate of 30 MHz. The HLT2 sequence, on the other hand, is run on a farm of CPU servers – a model that would be prohibitively costly for Upgrade II. Given the current evolution of processor performance, the baseline approach for Upgrade II is to perform the reconstruction algorithms of both HLT1 and HLT2 on GPUs. A strong R&D activity is also foreseen to explore alternative co-processors such as FPGAs and new emerging architectures.
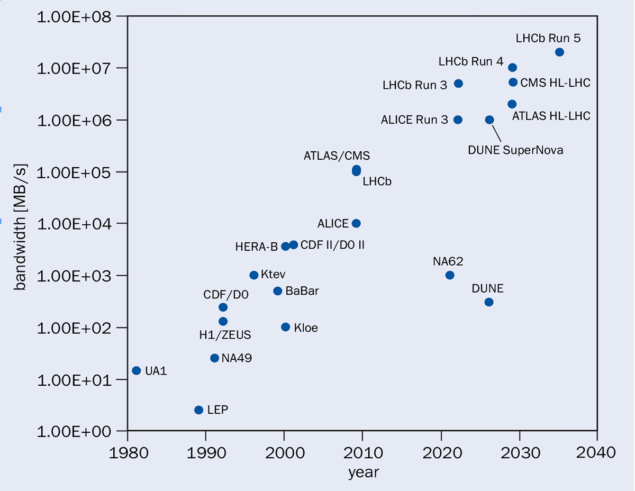
The second computing challenge for LHCb Upgrade II derives from detector simulations. A naive extrapolation from the computing needs of the current detector implies that 2.5 million cores will be needed for simulation in Run 5, which is one order of magnitude above what is available with a flat budget assuming a 10% performance increase of processors per year. All experiments in high-energy physics face this challenge, motivating a vigorous R&D programme across the community to improve the processing time of simulation tools such as GEANT4, both by exploiting co-processors and by parametrising the detector response with machine-learning algorithms.
Intimately linked with digital technologies today are energy consumption and efficiency. Already in Run 3, the GPU-based HLT1 is up to 30% more energy-efficient than the originally planned CPU-based version. The data centre is designed for the highest energy-efficiency, resulting in a power usage that compares favourably with other large computing centres. Also for Upgrade II, special focus will be placed on designing efficient code and fully exploiting efficient technologies, as well as designing a compact data acquisition system and optimally using the data centre.
A flavour of the future
The LHC is a remarkable machine that has already made a paradigm-shifting discovery with the observation of the Higgs boson. Exploration of the flavour-physics domain, which is a complementary but equally powerful way to search for new particles in high-energy collisions, is essential to pursue the next major milestone. The proposed LHCb Upgrade II detector will be able to accomplish this by exploring energy scales well beyond those reachable by direct searches. The proposal has received strong support from the 2020 update of the European strategy for particle physics, and the framework technical design report was positively reviewed by the LHC experiments committee. The challenges of performing precision flavour physics in the very harsh conditions of the HL-LHC are daunting, triggering a vast R&D programme at the forefront of technology. The goal of the LHCb teams is to begin construction of all detector components in the next few years, ready to install the new detector at the time of Long Shutdown 4.
Further reading
LHCb Collab. 2021 CERN-LHCC-2021-012.
LHCb Collab. 2018 CERN-LHCC-2018-027.