Despite tremendous efforts, the search for the constituents of dark matter has so far been unsuccessful. Interest is therefore growing in new experiments that probe dark-matter candidates such as axions and other very weakly interacting sub-eV particles.

Understanding the nature of dark matter is one of the most pressing problems in physics. This strangely nonreactive material is estimated, from astronomical observations, to make up 85% of all matter in the universe. The known particles of the Standard Model (SM) of particle physics, on the other hand, account for a paltry 15%.
Physicists have proposed many dark-matter candidates. Two in particular stand out because they arise in extensions of the SM that solve other fundamental puzzles, and because there are a variety of experimental opportunities to search for them. The first is the neutralino, which is the lightest supersymmetric partner of the SM neutral bosons. The second is the axion, postulated 40 years ago to solve the strong CP problem in quantum chromodynamics (QCD). While the neutralino belongs to the category of weakly interacting massive particles (WIMPs), the axion is the prime example of a very weakly interacting sub-eV particle (WISP).
Neutralinos as WIMPs have dominated the search for cold dark matter since the mid-1980s, when it was realised that massive particles with a cross section of the order of the weak interaction would result in precisely the right density to explain dark matter. There have been tremendous efforts to hunt for WIMPs both at hadron colliders, especially now at CERN’s Large Hadron Collider (LHC), and in large underground detectors, such as CDMS, CRESST, DARKSIDE, LUX, PandaX and XENON. However, up to now, no WIMP has been observed (CERN Courier July/August 2018 p9).

Very light bosons as WISPs are a firm prediction of models that solve problems of the SM by the postulation of a new symmetry which is broken spontaneously in the vacuum. Such extensions contain an additional scalar field with a potential shaped like a Mexican hat – similar to the Higgs potential in the SM (figure 1). This leads to spontaneous breaking of symmetry at a scale corresponding to the radius of the trough of the hat: excitations in the direction along the trough correspond to a light Nambu–Goldstone (NG) boson, while the excitation in the radial direction perpendicular to the trough corresponds to a heavy particle with a mass determined by the symmetry-breaking scale. The strengths of the interactions between such light bosons and regular SM particles are inversely proportional to the symmetry-breaking energy scale and are therefore very weak. Being light, very weakly interacting and cold due to their non-thermal production history, these particles qualify as natural WISP cold dark-matter candidates.
Primordial production
In fact, WISP dark matter is inevitably produced in the early universe. When the temperature in the primordial plasma drops below the symmetry-breaking scale, the boson fields are frozen at a random initial value in each causally-connected region. Later, they relax towards the minimum of their potential at zero fields and oscillate around it. Since there is no significant damping of these field oscillations via decays or interactions, the bosons behave as a very cold dark-matter fluid. If symmetry breaking occurs after the likely inflationary-expansion epoch of the universe (corresponding to a post-inflationary symmetry-breaking scenario), WISP dark matter would also be produced by the decay of topological defects from the realignment of patches of the universe with random initial conditions. A huge region in parameter space spanned by WISP masses and their symmetry-breaking scales can give rise to the observed dark-matter distribution.
The axion is a particularly well-motivated example of a WISP. It was proposed to explain the results of searches for a static electric dipole moment of the neutron, which would constitute a CP-violating effect of QCD. The size of this CP-violation, parameterised by the angle θ, is predicted to have an arbitrary value between –π and π, yet experiments show its absolute value to be less than 10–10. If θ is replaced by a dynamical field, θ(x), as proposed by Peccei and Quinn in 1977, QCD dynamics ensures that the low-energy effective potential of the axion field has an absolute minimum at θ = 0. Therefore, in vacuum, the CP violating effects due to the θ angle in QCD disappear – providing an elegant solution to the strong CP problem. The axion is the inevitable particle excitation of θ(x), and its mass is determined by the unknown breaking scale of the global symmetry.
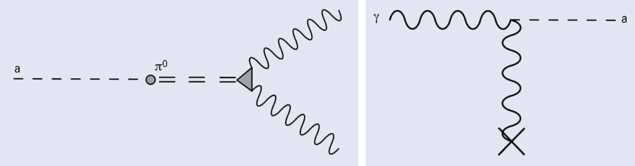
Lattice-QCD calculations performed last year precisely determined the temperature and corresponding time after the Big Bang when axion cold dark-matter could have formed as a function of the axion mass. It was found that, in the post-inflationary symmetry breaking scenario, the axion mass has to exceed 28 μeV; otherwise, the predicted amount of dark matter overshoots the observed amount. Taking into account the additional production of axion dark-matter from the decay of topological defects, an axion with a mass between 30 μeV and 10 meV may account for all of the dark matter in the universe. In the pre-inflationary symmetry breaking scenario, smaller masses are also possible.
Axions are not the only WISP species that could account for dark matter. There could be axion-like particles (ALPs), which are very similar to axions but do not solve the CP problem of QCD, or lightweight, weakly interacting, so-called hidden photons, for example. String theory suggests a plenitude of ALPs, which could have couplings to photons, leptons or light quarks.
Due to their tiny masses, WISPs might also be produced inside stars or alter the propagation of photons in the universe. Observations of stellar evolutions hint at such signals: red giants, helium-burning stars and white dwarfs seem to be experiencing unseen energy losses exceeding those expected from neutrino emission. Intriguingly, these anomalies can be explained in a unified manner by the existence of a sub-keV-mass axion or ALP with a coupling both to electrons and photons. Additionally, observations suggest that the propagation of TeV photons in the universe suffers less than expected from interactions with the extragalactic background light. This, in turn, could be explained by the conversion of photons into ALPs and back in astrophysical magnetic fields, interestingly with about the same axion–photon coupling strength as indicated by the observed stellar anomalies. Both effects have been known for almost 10 years. They are scientifically disputed, but a WISP explanation has not yet been excluded.
Experimental landscape
Most experiments searching for WISPs exploit their possible mixing with photons. Given the small masses and feeble interactions of axions and ALPs, however, building experiments that are sensitive enough to detect them is a considerable challenge. In the 1980s, Pierre Sikivie of the University of Florida in the US suggested a way forward based on the conversion of axions to photons: in a static magnetic field, the axion can “borrow” a virtual photon from the field and turn into a real photon (figure 2). Most experiments search for axions and ALPs in this way, with three main approaches being pursued: haloscopes, which look directly for dark-matter WISPs in the galactic halo of our Milky Way; helioscopes, which search for ALPs or axions emitted by the Sun; and laboratory experiments, which aim to generate and detect ALPs in a single setup.

Direct axion dark-matter searches differ in two aspects from WIMP dark-matter searches. First, axion dark matter would convert to photons, while WIMPs are scattered off matter. Second, the particle-number density for axion dark-matter, due to its low mass, is about 15 orders of magnitude larger than it is for WIMP dark matter. In fact, cold dark-matter axions and ALPs behave like a highly degenerate Bose–Einstein condensate with a de Broglie wavelength of the order of metres or kilometres for μeV and neV masses, respectively. Dark-matter axions and ALPs are thus much better pictured as a classical-field oscillation. In a magnetic field, they induce tiny electric-field oscillations with a frequency determined by the axion mass. If the de Broglie wavelength of the dark-matter axion is larger than the experimental setup, the tiny oscillations are spatially coherent in the experiment and can, in principle, be “easily” detected using a resonant microwave cavity tuned to the correct but unknown frequency. The sensitivity of such an experiment increases with the magnetic field strength squared, the volume of the cavity and its quality factor. Unfortunately, since the range of axion mass predicted by theories is huge, methods are required to tune the cavity to the frequency range corresponding to the respective axion masses.
This cavity approach has been the basis of most searches for axion dark-matter in the past decades, in particular the Axion Dark Matter Experiment (ADMX) at the University of Washington, US. Using a tuning rod inside the cavity to change the resonance frequency and, recently, by reducing noise in its detector system, the ADMX team has shown that it can reach axion dark-matter sensitivity. ADMX, which has been pioneering the field for two decades, is currently taking data and could find dark-matter axions at any time, provided the axion mass lies in the range 2–10 μeV. Meanwhile, the HAYSTAC collaboration at Yale University has very recently demonstrated that the same experimental approach can be expanded up to an axion mass of around 30 μeV. Since smaller-volume cavities (usually with lower quality factors) are needed to probe higher frequencies, however, the single-cavity approach is limited to axion masses below about 40 μeV. One novel method to probe higher masses is to use multiple matched cavities, as for example followed by the ADMX and the South Korean Center for Axion and Precision Physics.
Transitions
A different way to exploit the tiny electric-field oscillations from dark-matter axions in a strong magnetic field is to use transitions between materials with different dielectric constants: at surfaces, the axion-induced electromagnetic oscillations have a discontinuity, which is to be balanced by radiation from the surface. For a mirror with a surface area of 1 m² in a 10 T field, this would lead to an undetectable emission of around 10–27 W if axions make up all of the dark matter. Furthermore, the emission power does not depend on the axion mass. In principle, if a parabolic mirror with a surface area of 10,000 m² could be magnetised with a 10 T field, the predicted radiation power (10–23 W) could be focused and detected using state-of-the-art amplification techniques, but such an experiment seems impractical at present.
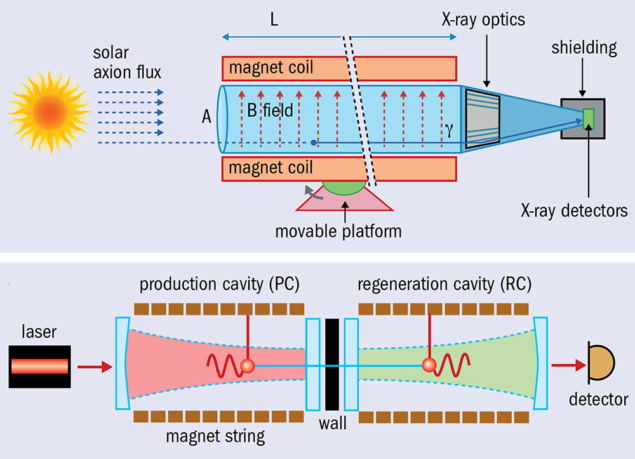
Alternatively, many magnetised dielectric discs in parallel can be placed in front of a mirror (figure 3): since the emission from all surfaces is coherent, constructive interference can boost the signal sensitivity for a given frequency range determined by the spacing between the discs. First studies performed in the past years at the Max Planck Institute for Physics in Munich have revealed that, for axion masses around 100 μeV, the sensitivity could be good enough to cover the predicted dark-matter axion mass range. The MADMAX (Magnetized Disc and Mirror Axion Experiment) collaboration, formed in October 2017, aims to use this approach to close the sensitivity gap in the well-motivated range for dark-matter axions with masses around 100 μeV. First design studies indicate that it is feasible to build a dipole magnet with the required properties using established niobium-titanium superconductor technology. As a first step, a prototype experiment is planned consisting of a booster with a reduced number of discs installed inside a prototype magnet. The experiment will be located at DESY in Hamburg, and first measurements sensitive to new ALPs parameter ranges are planned within the next few years.
Model independent searches
These direct searches for axion dark matter are very promising, but they are hampered at present by the unknown axion mass and rely on cosmological assumptions. Other, less-model dependent, experiments are required to further probe the existence of ALPs.
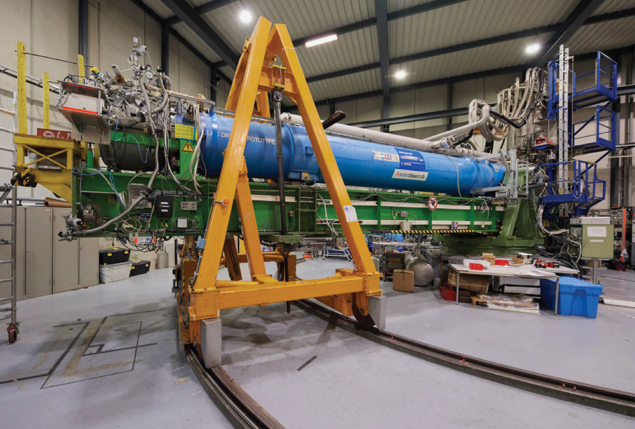
ALPs with energies of the order of a few keV could be produced in the solar centre, and could be detected on Earth by pointing a strong dipole magnet at the Sun: axions entering the magnet could be converted into photons in the same way they are in cavity experiments. The difference is that the Sun would emit relativistic axions with an energy spectrum very similar to the thermal spectrum in its core, so experiments need to detect X-ray photons and are sensitive to axion masses up to a maximum depending on the length of the apparatus (figure 4, top). This helioscope technique was brought to the fore by the CERN Axion Solar Telescope (CAST), shown in figure 5, which began operations in 2002 and has excluded axion masses above 0.02 eV. As a successor, the International Axion Observatory (IAXO) was formally founded in July 2017 and received an advanced grant from the European Research Council earlier this year. The near-term goal of the collaboration is to build a scaled-down prototype version of the experiment, called babyIAXO, which is under discussion for possible location at DESY.
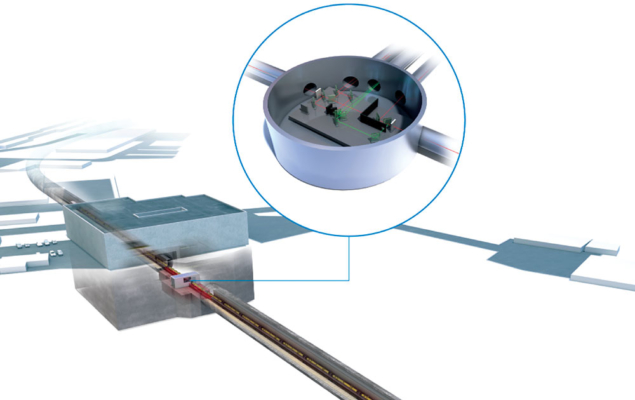
The third, laboratory-based, approach to search for WISPs also aims to generate and detect ALPs without any model assumption. In the first section of such an experiment, laser light is sent through a strong magnetic field so that ALPs might be generated via interactions of optical photons with the magnetic field. The second section is separated from the first one by a light-tight wall that can only be penetrated by ALPs. These would stream through a strong magnetic field behind the wall, allowing them to be re-converted into photons and giving the impression of light shining through a wall (figure 4, bottom).
Such experiments have been performed since the early 1990s, but no hint for any ALP has shown up. Today, the most advanced project in this laboratory-based category is ALPS II, currently being set up at DESY (figure 6). This experiment will use two optical resonators implemented into the apparatus to “recycle” the light before and increase the re-conversion probability of ALPs into photons behind the wall, allowing ALPS II to reach sensitivities beyond ALP–photon coupling limits from helioscopes. It also plans to use 20 dipoles from the former HERA collider, each of which has to be mechanically straightened, to generate the magnetic field.
Gaining momentum

Searches for very lightweight axions and ALPs, potentially explaining all of the dark matter around us, are strongly gaining momentum. CERN has been supporting such activities in the past (with solar-axion and dark-matter searches at CAST, and the OSQAR and CROWS experiments using the shining-light-through-walls approach) and is also involved in the R&D phase for next-generation experiments such as IAXO (CERN Courier September 2014 p17). With the new initiatives of MADMAX and IAXO, both of which could be located at DESY, and the ALPS II experiment under construction there, experimental axion physics in Europe is set to probe a large fraction of a well-motivated parameter space (figure 7). In addition to complementary experiments worldwide, the next 10 years or so should shine a bright light on WISPs as the solution to the dark-matter riddle, with thrilling data runs expected to start in the early 2020s.
Further reading
I Irastorza and J Redondo 2018 Prog. Part. Nucl. Phys. 102 89.
A Ringwald 2012 Phys. Dark Univ. 1 116.
M Kawasaki and K Nakayama 2013 Ann. Rev. Nucl. Part. Sci. 63 69.
P W Graham et al. 2015 Ann. Rev. Nucl. Part. Sci. 65 485.
A Caldwell et al. 2017 Phys. Rev. Lett. 118 091801.