The evolution of a key machine at CERN.

On 26 May 1972, the PS Booster (PSB) accelerated its first protons to the design energy of 800 MeV. The running-in team, led by Heribert Koziol, had prepared for this event by already sending beam from the 50 MeV Linac1 through the PSB injection line while the geometers were still busy aligning the ring magnets. Just five months later, the team succeeded in accelerating at half of the design intensity. This achievement was a great relief for the entire staff of the then Synchrotron Injector (SI) division, led by Giorgio Brianti and his deputy, Helmut Reich. However, the path to full intensity proved unexpectedly tough.
The concept of the PSB dates from the mid-1960s, when CERN’s 26 GeV Proton Synchrotron (PS) was getting into its stride and new and demanding clients – the Intersecting Storage Rings (ISR) and the Super Proton Synchrotron (SPS) – were on the horizon. By then, ideas to improve the performance of the PS by raising its output beam intensity from 1012 to 1013 protons per pulse (ppp) were already being considered.
Boosting intensity
Particles in synchrotrons suffer from resonances generated by residual imperfections of the magnetic guide (dipole) and focusing (quadrupole) fields, in particular for certain values of the “tunes” QH, QV (the number of horizontal/vertical oscillations per machine turn). Simple relations of the type mQH + nQV = p, with m and n small integers, are harmful “stop-bands” and must be avoided. In addition, high-intensity beams experience space charge, where the repulsive Coulomb force works against the external focusing and leads to a “Laslett tune-spread”, ΔQ. Studies at the PS (and also at the Alternating Gradient Synchrotron at Brookhaven) established that intensities leading to ΔQ > 0.25 (“space-charge limit”) cannot be digested at injection because they do not keep clear of stop-bands up to order |m| + |n| = 4. At higher (relativistic) energies, the repulsive force becomes weaker while the beam also gets stiffer – so that ΔQ shrinks with rising energy, scaling with 1/βγ2. Therefore, increasing the PS injection energy from 50 to 800 MeV would potentially boost its beam intensity by an order of magnitude.
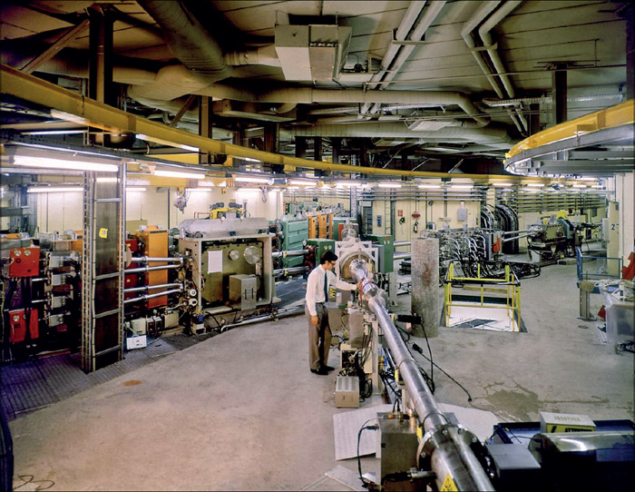
After a study led by the late Werner Hardt of several variants, including a 200 MeV linac and a rapid-cycling synchrotron, a slow-cycling 800 MeV booster synchrotron consisting of four superposed rings was adopted. Its injection energy would still be 50 MeV but each of the four rings could be filled up to the same space-charge limit as the whole PS ring, yielding a factor of 4. In addition, slow cycling would allow for longer bunches, yielding a further factor of 1.5. Hence, the new machine would accommodate – at 50 MeV – 1013 ppp rather than the 1.6 × 1012 ppp accelerated in the PS.
The four rings stacked on top of each other (figure 1), with a radius of 25 m (1/4 of the PS), consist of separate dipoles and quadrupoles (“separated function” magnets) – in contrast to the PS, which has “combined function” dipoles that incorporate gradients. Each of the 32 dipoles and 48 quadrupoles consists of a vertical stack of four magnets with a common yoke, enabling one main power supply to provide the current to all of them in series. An elaborate system of correction loops allows adjustment of the guide and focusing fields in each of the four rings. The 50 MeV beam from Linac1 is distributed vertically and multiturn-injected into each ring. Originally, the RF system for the PSB accelerated five bunches per ring (RF harmonic number h = 5) to an ejection energy of 800 MeV. After being synchronized, the five bunches were horizontally extracted and recombined vertically to form a string of 20 bunches, corresponding to the harmonic number of the PS’s RF system. The transverse optics (“lattice”) of the PSB ring, as well as the injection system and the recombination/transfer line (figure 2), were all designed by the late Claude Bovet.
Construction of the PSB started in 1968, with the centre of the machine lying exactly on the Swiss-French border. Many novel technological challenges had to be addressed, such as: unprecedented requirements on field quality and equality between the superposed magnet gaps; “kicker” magnets with rapid rise/fall times; and stable and reliable power converters operating directly from the grid. The ambitious aims for beam intensity and quality demanded special efforts for mechanical stability, beam diagnostics, vacuum equipment, radiation protection, assuring hands-on maintenance, and general reliability. Moreover, the PSB served as a “guinea pig” for the then innovative computer-control system aimed at monitoring all of the machine parameters.
Design intensity – and beyond
While the quick initial success of the running-in testified to the soundness of the basic choices and the high quality of the construction work, major difficulties later hampered the progress towards design performance. The first was a strong energy-jitter of the beam from Linac1, which was eventually stabilized at the expense of the beam current (50 mA instead of the 100 mA that was specified). With the help of experienced accelerator physicists, Jacques Gareyte and the late Frank Sacherer, the obstacles were addressed one by one. The “working point” (QH, QV) was moved from around (4.8, 4.8) to (4.2, 5.3), mitigating transverse beam blow-up caused by repeated stop-band crossing arising from the synchrotron motion of the protons within the bunch. Furthermore, a fast change of the working point during acceleration proved beneficial, profiting from the shrinking ΔQ (figure 3). Destructive coherent bunch-oscillations were stabilized by “Magnani shaking” and later by a coupled-bunch feedback system. A first pay-off came in 1973, when the search for neutral currents with the Gargamelle bubble chamber benefitted from the increased supply of protons from the PS with the PSB as injector. By 1974 the PS reached 1013 ppp – the design performance of the upgrade programme.

However, this is not the end of the story. By 1978, the new Linac2 – still at 50 MeV but with 150 mA beam current – replaced Linac1 and dramatically increased the PSB’s potential, although it took a couple of years to exploit this improvement fully. Installing multipole correctors to eliminate stop-bands allowed intensities with larger tune-spread to be accommodated. The addition of “bunch-
flattening cavities” in the PSB, fostered by the late George Nassibian, lowered the peak density of the bunches and thus ΔQ, enabling an intensity some 25% higher to be accepted. Fast feedback systems compensating unwanted excitations by the bunches on the cavities (“beam loading”), as well as transverse dampers, also proved beneficial, culminating in the PSB’s acceleration of 3 × 1013 ppp by 1985. Now the PS was at pains to digest this beam at 800 MeV, in particular the high-density proton bunches for antiproton production, which were obtained by simultaneously ejecting two PSB rings and adding the bunches vertically in the recombination line, almost doubling their line density. To cope with this, the PSB was promoted to a 1 GeV machine after minor hardware modifications, increasing the PS space-charge limit by a further 25%.
Experiments involving light ions became popular in the early 1980s and the old Linac1 was successfully converted to accelerate oxygen and sulphur ions, deuterons and alpha particles. The PSB followed suit. However, the issue now was not too high an intensity but one that was very low (by three orders of magnitude), which together with new acceleration frequencies challenged the beam diagnostics and RF systems. The low-intensity ion cycles had to be added to the supercycle – during which all beam parameters are modified from cycle to cycle to adapt to the requirements of the end-users. With the advent of a dedicated ion accelerator, Linac3, the PSB made its way up the periodic table to reach lead and, later, indium ions.
When CERN’s first accelerator – the venerable 600 MeV Synchrocyclotron, by then feeding the ISOLDE online separator with protons – came to the end of its life after 33 years of meritorious services, ISOLDE looked for a new source. Following the suggestion to use the PSB’s 1 GeV beam on the many spare cycles available, ISOLDE was relocated at the PSB in 1992: an experimental area of its own was the coming-of-age present for the Booster’s 20th anniversary.
Fit for the LHC
It is a CERN tradition that new machines use existing accelerators as injectors, and the LHC is no exception. Clearly, all of the accelerators of the proton-injector chain – Linac2–PSB–PS–SPS – had to undergo major upgrade programmes to be fit for the new machine. Among the requirements, the beam would have to fit into the tiny LHC aperture while having sufficient intensity to ensure high-luminosity operation. However, this implied a beam-brilliance at injection into the PSB that would lead to an unrealistic space-charge detuning of ΔQ up to 1. This could be reduced to a more acceptable 0.5 by two-batch filling of the PS, but only if each batch could be squeezed into one half of its circumference. Accelerating one rather than five bunches in each PSB ring and applying clever timing of the ejection/recombination kickers made this feasible, although the first batch in the PS has to dwell for 1.2 s on the 1 GeV “front porch”, proving vulnerable to space-charge effects.
A further improvement came from increasing the PSB–PS transfer energy to 1.4 GeV, reducing ΔQ in the PS to 0.2, well below the space-charge limit of 0.25, owing to the 1/βγ2 scaling. Upgrading a machine built for 800 MeV to 1.4 GeV was no minor task. It involved a new main power supply, increased water cooling and a partial renewal of the magnets and their power supplies in the transfer line to the PS. The rings had to be equipped with new h = 1 (2 MHz) cavities and as well as recycled h = 2 (4 MHz) cavities, the former accelerating one bunch in each ring, the latter for bunch flattening or accelerating two bunches a ring for some users. The lion’s share of this upgrade was provided by Canada as part of its contribution to the LHC. Installation of the new hardware in both the PSB and the PS was completed by early 2000 and after a short running-in period the PS complex demonstrated its capability to supply the beams required by the LHC.

Owing to its unique four-ring structure, the PSB is a versatile machine that can deliver beams of different energy, intensity, density, shape or time-structure to many users, cycle after cycle. These are grouped in supercycles of various lengths that are adapted to the operation programme. Just for the LHC, some 10 different beams were prepared and made ready to work, all of them with their own intensity (over the range 5 × 109 – 6.5 × 1012 ppp) and emittance characteristics. Other cycles produce up to 3.7 × 1013 protons for ISOLDE, around 2.5 × 1013 protons for the CERN Neutrinos to Gran Sasso project, 1.5 × 1013 protons with small emittances for the Antiproton Decelerator or intensities as low as 2 × 1011 protons for slow extraction from the PS. In particular, the versatility of the recombination line enables the bunches from the four rings to be furnished with different distances between them to satisfy the requirements of the users.
By around 2003, two modifications to beam optics were proposed and put into operation. First, the optics of the 50 MeV injection line were improved so that the dispersion and its derivative vanish at the injection point. This reduces the beam size and the losses in the last leg of the line (where the acceptance was limited) without perturbing the injection efficiency. Second, the working point of the machine was changed to avoid the systematic resonance 3QV = 16, which limited the performance of the outer rings 1 and 4. The quadrant (QH, QV) = (4.2, 4.3) instead of (4.2, 5.3) proved able to accommodate the enormous tune-spread ΔQ of 0.6 (using the same dynamic tune-change during the cycle) despite its apparent drawbacks: namely, the presence of the “Montague” coupling resonance 2QH – 2QV = 0 and the systematic resonances 4QH,V = 16. As a result, the PSB reached a record of 4.2 × 1013 protons accelerated, with the four rings having similar performances (figure 4).
By 2006, when construction of the LHC was in full swing, the operation teams of all of the accelerators moved to a common control room – the CERN Control Centre – to increase the operational efficiency of the LHC and its injector chain. For the PSB team, this meant a change in culture owing to the larger distance between the PS complex and the new control room. However, the merging of the teams proved invaluable for the running-in and operation of the LHC, which uses all of the prepared beams.
The future
The need for beams for the LHC with parameters even more demanding than what is provided today, together with the decision to operate the existing injector complex throughout the lifetime of the LHC, has triggered a major consolidation and upgrade project. As far as the PSB is concerned, the upgrade programme consists of two parts: modifications of the injection for 160 MeV charge-exchange injection from the new Linac4; and an energy upgrade of the PSB rings and extraction/transfer systems to 2 GeV.
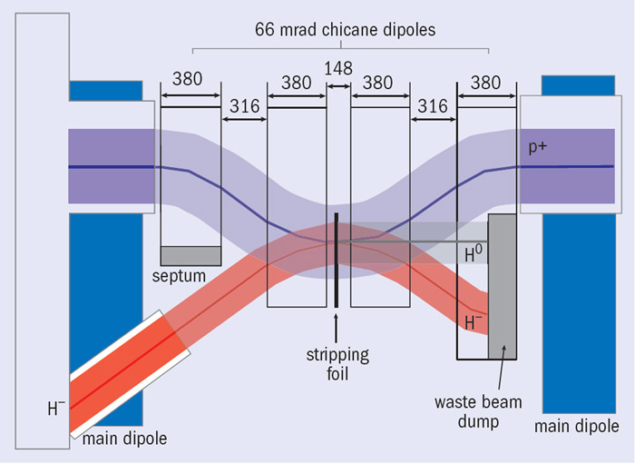
The benefits of switching from Linac2 (50 MeV protons) to Linac4 (160 MeV H–) are twofold. With the increase of beam energy from 50 to 160 MeV, the relativistic βγ2 factor increases by a factor of two – doubling the intensity that can be accumulated within a given emittance and hence beam brilliance (ratio intensity/emittance). The other significant benefit is expected from changing the injection scheme to charge-exchange injection (figure 5). While the current multiturn injection is associated with a beam loss of up to 50% of the intensity on the injection septum, the new scheme will essentially be loss-free (apart from a few per cent owing to the stripping efficiency). Moreover, the new injection scheme will make it possible to tailor emittances by means of “phase-space painting” according to the needs of individual users.
The aim of the further energy upgrade of the PSB to 2 GeV is to reduce space-charge effects at injection into the PS, removing once more this bottleneck in the LHC injector chain. This should increase the beam brilliance throughout the LHC injector chain so that the LHC can reach its ultimate luminosities. The expected gain can be deduced from the values of βγ2 at 2.0 GeV and 1.4 GeV; the factor of 1.63 corresponds to an intensity increase of 60% within given emittance values.
The upgrade to 2 GeV will be the third energy increase in the history of the PSB, having gone in steps from 800 MeV to 1 GeV and then to the current 1.4 GeV. The most important technical challenges will be the operation of the main magnets at field levels that are 30% higher than those at 1.4 GeV, together with the replacement of the main power supply, as well as the upgrade of the extraction and recombination system. Also, many components need modification or replacement to operate in the new parameter range. Following completion of the upgrade, the PSB will – in many parts – be a new machine, without losing its current versatility.
In its 40-year history, the PSB has undergone several upgrades and is today operating with its highest availability and flexibility, and far beyond its original design specifications. The ongoing consolidation and upgrade programme aims to operate the PSB throughout the lifetime of the LHC. This will ensure that it remains one of CERN’s backbone accelerators for the foreseeable future.