A key observation at the LHC marks a major milestone in a 30-year long journey.

Since its birth, the Standard Model of particle physics has proved to be remarkably successful at describing experimental measurements. Through the prediction and discovery of the W and Z bosons, as well as the gluon, it continues to reign. The recent discovery of a Higgs boson with a mass of 126 GeV by the ATLAS and CMS experiments indicates that the last piece of this jigsaw puzzle has been put into place. Yet, despite its incredible accuracy, the Standard Model must be incomplete: it offers no explanation for the cosmological evidence of dark matter, nor does it account for the dominance of matter over antimatter in the universe. The quest for what might lie beyond the Standard Model forms the core of the LHC physics programme, with ATLAS and CMS systematically searching for the direct production of a plethora of new particles that have been predicted by various proposed extensions to the model.
Complementary methods
As a consequence of its excellent performance – including collisions at much higher energies than previously achieved and record integrated luminosities – the LHC also provides complementary and elegant approaches to finding evidence of physics beyond the Standard Model, namely precision measurements and studies of rare decays. Through Heisenberg’s uncertainty principle, quantum loops can appear in the diagrams that describe Standard Model decays, which are influenced by particles that are absent from both the initial and final states. This experimentally well established general concept opens a window to observe the effects of undiscovered particles or of other new physics in well known Standard Model processes. Because these effects are predicted to be small, the proposed new-physics extensions remain consistent with existing observations. Now, the high luminosity of the LHC and the unprecedented precision of the experiments are allowing these putative effects to be probed at levels never before reached in previous measurements. Indeed, this is the prime field of study of the LHCb experiment, which is dedicated to the precision measurement of decays involving heavy quarks, beauty (b) and charm (c). The general-purpose LHC experiments can also compete in these studies, especially where the final states involve muons.
Behind the seemingly simple decay topology hides a tricky experimental search aimed at finding a few signal events in an overwhelming background
A rare confluence of factors makes the decay of beauty mesons into dimuon (μ+μ–) final states an ideal place to search for this sort of evidence for physics beyond the Standard Model. The decays of B0 (a beauty antiquark, b, and a down quark, d) and Bs (a b and a strange quark, s) to μ+μ? are suppressed in the Standard Model yet several proposed extensions predict a significant enhancement (or an even stronger suppression) of their branching fractions. A measurement of the branching fraction for either of these decays that is inconsistent with the Standard Model’s prediction would be a clear sign of new physics – a realization that sparked off a long history of searches. For the past 30 years, a dozen experiments at nearly as many particle colliders have looked for these elusive decays and established limits that have improved by five orders of magnitude as the sensitivities approach the values predicted by the Standard Model (figure 2). Last November, LHCb found the first clear evidence for the decay Bs → μμ, at the 3.5σ level. Now both the CMS and LHCb collaborations have updated their results for these decays.
Behind the seemingly simple decay topology hides a tricky experimental search aimed at finding a few signal events in an overwhelming background: only three out of every thousand million Bs mesons are expected to decay to μμ, with the rate being even lower for the B0. The challenge is therefore to collect a huge data sample while efficiently retaining the signal and filtering out the background.
Several sources contribute to the large background. B hadrons decay semi-leptonically to final states with one genuine muon, a neutrino and additional charged tracks that could be misidentified as muons, therefore mimicking the signal’s topology. Because the emitted neutrino escapes with some energy, these decays create a dimuon peak that is shifted to a lower mass than that of the parent particle. The decays Λb → pμν form a dangerous background of this kind because the Λb is heavier than the B mesons, so these decays can contribute to the signal region. Two-track hadronic decays of B0 or Bs mesons also add to the background if both tracks are mistaken for muons. This “peaking background” – fortunately rare – is tricky because it exhibits a shape that is similar to that which is expected for the signal events. The third major background contribution arises from events with two genuine muons produced by unrelated sources. This “combinatorial” background leads to a continuous dimuon invariant-mass distribution, overlapping with the B0 and Bs mass windows, which is reduced by various means as discussed below.
The first hurdle to cross in finding the rare signal events is to identify potential candidates during the bursts of proton–proton collisions in the detectors. Given the peak luminosities reached in 2012 (up to 8 × 1033 cm–2 s–1), the challenge for CMS was to select by fast trigger the most interesting 400 events a second for recording on permanent storage and prompt reconstruction, with around 10 per second reserved for the B → μμ searches. With its smaller event size, LHCb could afford a higher output rate from its trigger, recording several kilohertz with a significant fraction dedicated to dimuon signatures.
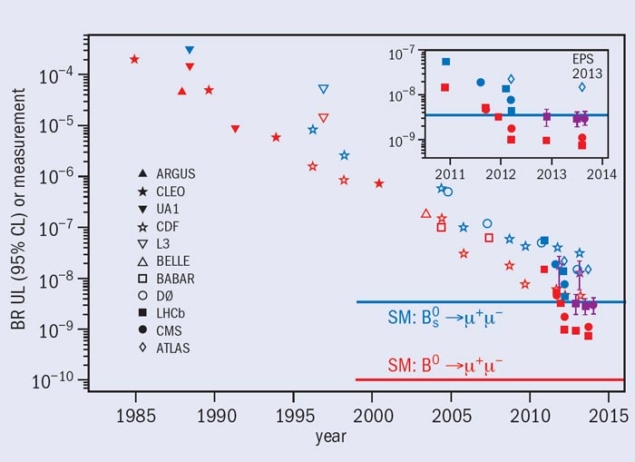
The events selected by the trigger are then filtered according to the properties of the two reconstructed muons to reject as much background as possible while retaining as many signal events as possible. In particular, hadrons misidentified as muons are suppressed strongly through stringent selection criteria applied on the number of hits recorded in the tracking and muon systems, on the quality of the track fit and on the kinematics of the muons. In LHCb, information from the ring-imaging Cherenkov detectors further suppresses misidentification rates. Additional requirements ensure that the two oppositely charged muons have a common origin that is consistent with being the decay point of a (long-lived) B meson. The events are also required to have candidate tracks that are well isolated from other tracks in the detector, which are likely to have originated from unrelated particles or other proton–proton collisions (pile-up). This selection is made possible by the precise measurements of the momentum and impact parameter provided by the tracking detectors in both experiments. The good dimuon-mass resolution (0.6% at mid-rapidity for CMS and 0.4% for LHCb) limits the amount of combinatorial background that remains under the signal peaks. Figure 1 shows event displays from the two experiments, each including a displaced dimuon compatible with being a B → μμ decay.
The final selection of events in both experiments is made with a multivariate “boosted decision tree” (BDT) algorithm, which discriminates signal events from background by considering many variables. Instead of applying selection criteria independently on the measured value of each variable, the BDT combines the full information, accounting for all of the correlations to maximize the separation of signal from background. CMS applies a loose selection on the BDT discriminant to ensure a powerful background rejection at the expense of a small loss in signal efficiency. Both experiments categorize events in bins of the BDT discriminant. LHCb has a higher overall efficiency, which together with the larger B cross-section in the forward region compensates for the lower integrated luminosity, so the final sensitivity is similar for both experiments.
The observable that is sensitive to potential new-physics contributions is the rate at which the B0 or Bs mesons decay to μμ, which requires a knowledge of the total numbers of B0 and Bs mesons that are produced. To minimize measurement uncertainties, these numbers are evaluated by reconstructing events where B mesons decay through the J/ψK channel, with the J/ψ decaying to two muons. This signature has many features in common with the signal being sought but has a much higher and well known branching fraction. The last ingredient required is the fraction of Bs produced relative to B+ or B0 mesons, which LHCb has determined in independent analyses. This procedure provides the necessary “normalization” without using the total integrated luminosity or the beauty production cross-section. LHCb also uses events with the decay B0 → K+π– to provide another handle on the normalization.
Results
Both collaborations use unbinned maximum-likelihood fits to the dimuon-mass distribution to measure the branching fractions. The combinatorial background shape in the signal region is evaluated from events observed in the dimuon-mass sidebands, while the shapes of the semileptonic and peaking backgrounds are based on Monte Carlo simulation and are validated with data. The magnitude of the peaking background is constrained from measurements of the fake muon rate using data control samples, while the levels of semileptonic and combinatorial backgrounds are determined from the fit together with the signal yields.

Both collaborations use all good data collected in 2011 and 2012. For CMS, this corresponds to samples of 5 fb–1 and 20 fb–1, respectively, while for LHCb the corresponding luminosities are 1 fb–1 and 2 fb–1. The data are divided into categories based on the BDT discriminant, where the more signal-like categories provide the highest sensitivity. In the fit to the CMS data, events with both muons in the central region of the detector (the “barrel”) are separated from the others (the “forward” regions). Given their excellent dimuon-mass resolution, the barrel samples are particularly sensitive to the signal. All of the resulting mass distributions (12 in total for CMS and eight for LHCb) are then simultaneously fit to measure the B0 → μμ and Bs → μμ branching fractions, yielding the results that are shown in figure 3.
For both experiments, the fits reveal an excess of Bs → μμ events over the background-only expectation, corresponding to a branching fraction BF(Bs → μμ) = 3.0+1.0–0.9 × 10–9 in CMS and 2.9+1.1–1.0 × 10–9 in LHCb, where the uncertainties reflect statistical and systematic effects. These measurements have significances of 4.3σ and 4.0σ, respectively, evaluated as the ratio between the likelihood obtained with a free Bs → μμ branching fraction and that obtained by fixing BF(Bs → μμ) = 0. The results have been combined to give BF(Bs → μμ) = 2.9±0.7 × 10–9 (CMS+LHCb).
Both CMS and LHCb reported this long-sought observation at the EPS-HEP conference in Stockholm in July and in back-to-back publications submitted to Physical Review Letters (CMS collaboration 2013, LHCb collaboration 2013).
The combined measurement of Bs → μμ by CMS and LHCb is consistent with the Standard Model’s prediction, BF(Bs → μμ) = 3.6±0.3 × 10–9, showing that the model continues to resist attempts to see through its thick veil. The same fits also measure the B0 → μμ branching fraction. They reveal no significant evidence of this decay and set upper limits at the 95% confidence level of 1.1 × 10–9 (CMS) and 0.74 × 10–9 (LHCb). These limits are also consistent with the Standard Model, although the measurement fails to reach the precision required to probe the prediction.
While the observation of a decay that has been sought for so long and by so many experiments is a thrilling discovery, it is also a bittersweet outcome. Much of the appeal of the Bs → μμ decay-channel was in its potential to reveal cracks in the Standard Model – something that the measurement has so far failed to provide. However, the story is far from over. As the LHC continues to provide additional data, the precision with which its experiments can measure these key branching fractions will improve steadily and increased precision means more stringent tests of the Standard Model. While these results show that deviations from the expectations cannot be large, even a small deviation – if measured with sufficient precision – could reveal physics beyond the Standard Model.
Additionally, the next LHC run will provide the increase in sensitivity that the experiments need to measure B0 → μμ rates at the level of the Standard Model’s prediction. New physics could be lurking in that channel. Indeed, the prediction for the ratio of the Bs → μμ and B0 → μμ decay rates is well known, so a precise measurement of this quantity is a long-term goal of the LHC experiments. And even in the scenario where the Standard Model continues its undefeated triumphant path, theories that go beyond it must still describe the existing data. Tighter experimental constraints on these branching fractions would be powerful in limiting the viable extensions to the Standard Model and could point towards what might lie beyond today’s horizon in high-energy physics. With the indisputable observation of Bs → μμ decays, experimental particle physics has reached a major milestone in a 30-year-long journey. This refreshing news motivates the LHC experimental teams to continue forward into the unknown.