Impressive headway in the study of double beta decay.
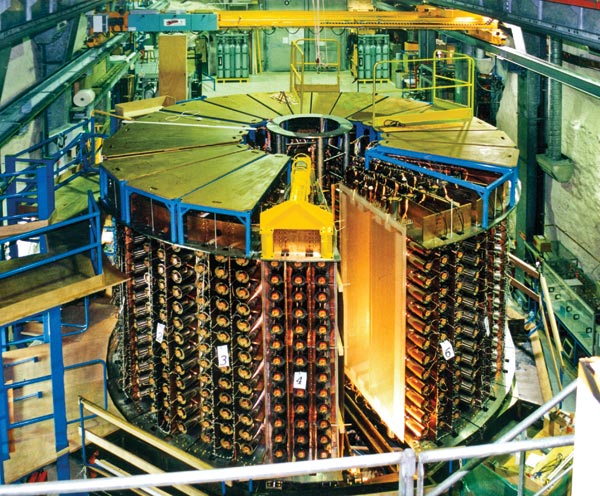
Image credit: CNRS and CEA.
Located under 1700 m of rock in the Modane Underground Laboratory (LSM) at the middle of the Fréjus Rail Tunnel, the NEMO 3 experiment was designed to search for neutrinoless double beta decay, with the aim of discovering the nature of the neutrino – whether it is a Majorana or Dirac particle – and measuring its mass. The experiment ran for seven years before it finally stopped taking data in January 2010. While the sought-after decay mode remained elusive, NEMO 3 nevertheless made impressive headway in the study of double beta decay, providing new limits on a number of processes beyond the Standard Model.
Standard double beta decay (ββ2ν) involves the simultaneous disintegration of two neutrons in a nucleus into two protons with the emission of two electrons accompanied by two antineutrinos, (A,Z) → (A,Z+2) + 2e– +2ν. It is a second-order Standard Model process and for it to occur the transition to the intermediate nucleus accessible by normal beta decay, (A,Z) → (A,Z+1) + e– + ν, must be forbidden by conservation of either energy or angular momentum. In nature, there are 70 isotopes that can decay by ββ2ν and experiments have observed this process in 10 of these, with half-lives ranging from 1018 to 1021 years. However, ββ2ν decay is not sensitive to the nature or mass of the neutrino, unlike double beta decay with no emitted neutrinos (ββ0ν). This process, (A,Z) → (A,Z+2) + 2e–, is forbidden by the Standard Model electroweak interaction because it violates the conservation of lepton number (ΔL = 2). Such a decay can occur only if the neutrino is a Majorana particle (a fermion that is its own antiparticle). Non-Standard Model processes that can lead to ββ0ν decay include the exchange of a light neutrino, in which case the inverse of the ββ0ν half-life depends on the square of the effective neutrino mass. Other possible processes involve a right-handed neutrino current, a Majoron coupling or supersymmetric particle exchange.
The experimental signature for double beta-decay processes appears in the sum of the energy of the two electrons. For ββ0ν decay, this would have a peak at the Qββ transition energy (typically 2–4 MeV), while for ββ2ν decay it takes the form of a continuous spectrum from zero to Qββ. There are also two other observables: the angular distribution between the two electrons and the individual energy of the electrons. These two variables can distinguish which process is responsible for ββ0ν decay, if it is observed.
The NEMO collaboration – where NEMO stands for the Neutrino Ettore Majorana Observatory – has been working on ββ0ν decay since 1989. The design of the NEMO 3 detector, which evolved from two prototypes, NEMO 1 and NEMO 2, began in 1994 and construction started three years later. The method uses a number of thin source foils of enriched double beta-decay emitters surrounded by two tracking volumes and a calorimeter.
The challenge for any search for ββ0ν decay is the control of the backgrounds from cosmic rays, natural radioactivity, neutrons and radon. The background comes from any particle interactions or radioactive decays that can produce two electrons in the source foils. Because the signal level is so low, even third- and fourth-order processes can be a problem. Cosmic rays are suppressed by installing the experiment in a deep underground laboratory, as at the LSM. Natural radioactivity is reduced by material selection and purification of the source isotopes: the source foils in NEMO 3 had a radioactivity level a million times less than the natural level of radioactivity (around 100 Bq/kg). Neutrons and high-energy γ-rays are suppressed by specially designed and adapted shielding.
The NEMO 3 detector
The principle of NEMO 3 was to detect the two emitted electrons and to measure their energy as well as their angular distribution and their individual energies. The identification of the electrons reduces drastically the background compared with the calorimetric techniques of other experiments. The price of this advantage is a rather modest energy resolution, partly as a result of the electron’s energy loss in the source foils. However, the experimental sensitivity for ββ0ν depends on the product of the energy resolution and the number of background events. The source foils in NEMO 3 had a thickness of around 100 μm, which corresponded to a compromise between the amount of radioactive isotope and the electrons’ energy losses.
Another advantage of this experimental technique is the possibility of using different isotopes. The double beta-decay source inside NEMO 3 had a total mass of 10 kg, which was shared as follows: 6.914 kg of 100Mo, 0.932 kg of 82Se, 0.405 kg of 116Cd, 0.454 kg of 130Te, 37.0 g of 150Nd, 9.4 g of 96Zr and 7.0 g of 48Ca. These isotopes were enriched in Russia. In addition, two ultrapure sources of copper (0.621 kg) and natural tellurium (0.491 kg) were used to measure the external background. It is the first time that a detector has measured seven different double beta-decay emitters at the same time.
The NEMO 3 detector was made of 20 identical sectors. The tracking volume consisted of 8000 drift chambers working in Geiger mode. The volume was filled with a mixture of helium, 4% alcohol, 1% argon and a few parts per million of water to ensure the stable behaviour of the chamber. Electrons could be tracked with energy down to 100 keV with an efficiency of greater than 99%.The calorimeter was made of 2000 plastic scintillators coupled to low-radioactivity Hamamatsu phototubes. The choice of plastic scintillator was driven by the low Z to reduce back scattering, the low radioactivity and the cost. The calorimeter allowed measurements of both the energy (σ=3.6% at 3 MeV) and the time of flight (σ= 300 ps at 1 MeV).
A coil created a magnetic field of 0.003 T to enable the identification of the sign of the electrons. The shielding was made of 20 cm of iron to reduce γ-ray background and 30 cm of water to reduce the neutron background. A tent flushed with air containing just 15 mBq/m3 of radon surrounded the whole detector.

The unique feature of the NEMO 3 experiment was its ability to identify electrons, positrons, γ-rays and delayed α-particles. Figure 2 shows a typical double beta-decay event in NEMO 3 with two electrons emitted from a source foil, with the track curvature in the magnetic field identifying the charge and the struck scintillator blocks measuring the energy and the time of flight. The timing is important to distinguish a background electron crossing the detector (Δt=4 ns) from two electrons coming from a source foil (Δt=0 ns).
The experiment has measured the background through various analysis channels: single e–, e–+γ, e+α, e–+α+γ, e–+γ+γ, e–+e+ and so on. This allows measurements to be made of the actual backgrounds from residual contamination of the source foils as well as from the surrounding materials. Figure 3 demonstrates the ability of the experiment to identify the many sources of external background in the e–γ channel (as an example) for the 100Mo source foil.
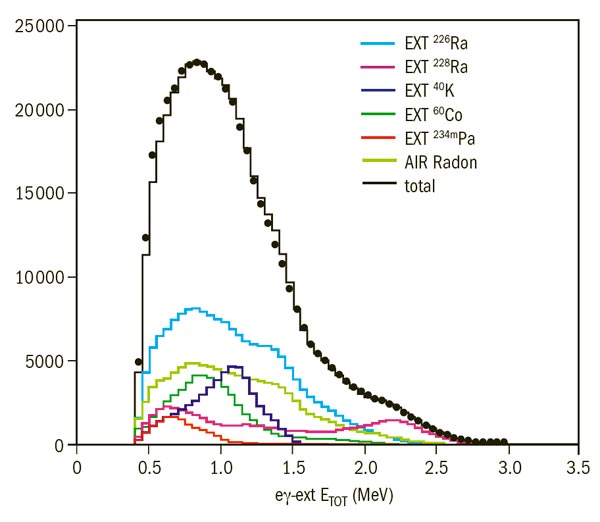
NEMO 3 has produced an impressive list of results. The main result is, of course, related to the search for ββ0ν decay. Figure 4 shows the sum of the electron energy for 7 kg of 100Mo after 4.5 years of data-taking, zoomed into the region where the signal for ββ0ν decay is expected. The measurement of all of the kinematic parameters and the identification of all of the sources of background allows a 3D likelihood analysis to be performed. The result is a limit on the half-life of T1/2 > 1×1024 years, corresponding to a neutrino mass limit <mν> < 0.3–0.9 eV. The range corresponds to the spread associated with the different nuclear matrix-element calculations that must be used to extract the effective neutrino mass. This limit obtained with 7 kg of 100Mo is one of the best limits, together with the result of <mν> <0.3 – 0.7 eV from the Cuoricino experiment (12 kg of 130Te) and of <mν> < 0.3–1.0 eV from the Heidelberg-Moscow experiment (11 kg of 76Ge).

One possible scenario for ββ0ν involves the emission of the Majoron, the hypothetical massless boson associated with the spontaneous breaking of baryon-number minus lepton-number (B-L) symmetry. NEMO 3 has obtained the best limit so far for the Majoron-neutrino coupling, with gM < (0.4–1.8) × 10–4. The experiment has also set a limit on the λ parameter in models where a right-handed current exists for neutrinos, with λ < 1.4 × 10–6. These limits were obtained by analysing the angular distributions of the decay electrons and they are therefore unique to NEMO 3.
In addition, NEMO 3 has measured the half-lives for seven ββ2ν decays, providing a high-precision test of the Standard Model and nuclear data that can be used in theoretical calculations. In seven years, more than 700,000 events were recorded for ββ2ν emission from 100Mo. Figure 5 shows the energy spectrum, angular distribution and single energies measured for 100Mo. The first direct detection of ββ2ν decay to the 0+ excited state has also been measured for this nucleus and the first limit on the bosonic component of the neutrino has been obtained.
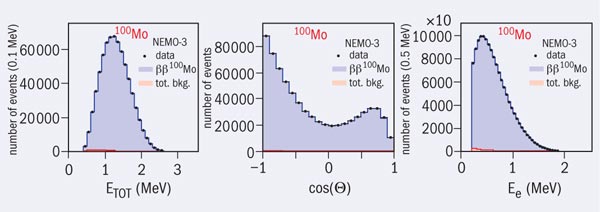
The NEMO 3 detector has demonstrated a powerful method for searching for neutrinoless double beta decay, with the unique capability of measuring all kinematic parameters of the decay. The next step for the NEMO collaboration is to build the SuperNEMO detector, which will accommodate 100 kg of source foil (82Se, 150Nd or 48Ca) to reach a sensitivity of 50 meV on the effective mass of the neutrino. A demonstrator module is under construction in several laboratories around the world and will start operation in 2013 in the LSM, with 7 kg of 82Se. The main improvement in this larger detector over NEMO 3 will be the energy resolution (σ=1.7% at 3 MeV) and the reduction of the background by a factor of 10. This demonstrator will improve the current limit on the effective neutrino mass and is expected to reach the goal of a zero-background experiment for 7 kg of source and two years of data-taking, which has never been done before. With this demonstration, the collaboration will be ready to build more Super NEMO modules up to the maximum source mass possible.
• The NEMO and SuperNEMO collaboration is formed by laboratories from France, the UK, Russia, the US, Japan, the Czech Republic, Slovakia, Ukraine, Chile and Korea. The LSM is operated by the CNRS and the CEA.