An observed excess of diffuse gamma rays traces the distribution of dark matter in our galaxy through its annihilations. Wim de Boer describes a recent analysis of the data.
It is well known that visible matter in the form of stars and galaxies makes up only a small fraction of the total energy in our universe. The latest evidence is that 5% is made from particles we know about, while 95% is in a form we know nothing about. The large non-visible, “dark” fraction is known to exist from its gravitational effects and comes in two forms: dark matter, constituting 23% of the total energy, provides the familiar gravitational pull, thus slowing down the expansion of the universe; the remainder, the dominant 72% of the total energy, causes antigravity, i.e. it accelerates the expansion of the universe.
Dark matter was so named by the Swiss scientist Fritz Zwicky. In studying the movements of the galaxies in the Coma cluster in the 1930s, he discovered that there must be much more matter than is visible. Later, the rotation speeds of gases and stars in spiral galaxies revealed that practically every galaxy has a halo of dark matter surrounding it. This dark matter must be much more widely distributed than the visible matter, since the rotation speeds do not fall off like 1⁄√r, as expected from the visible matter in the centre, but stay more or less constant.
The fact that the dark matter is distributed over large distances implies that it undergoes little energy loss, so any interactions it has must be weak. Therefore, dark-matter particles are generically called WIMPs, for weakly interacting massive particles. These WIMPs must, however, be able to annihilate if they were produced in thermal equilibrium with all other particles in the early universe. At that time the number densities of different particles were all of the same order of magnitude and just as the baryon/photon ratio was reduced by 10 orders of magnitude by baryon annihilation, the WIMP number density, which is of the same order of magnitude as the baryon number density, can only have been reduced by annihilation, assuming the WIMPs are stable. (If they are not stable they must have a lifetime of the order of the lifetime of the universe, otherwise they would no longer exist.)
The gamma rays play a very special role as they point straight back to the source
If WIMPs in our galaxy collide and annihilate into quark pairs, these in turn will produce stable particles including gamma rays. The gamma rays play a very special role as they point straight back to the source, in contrast to charged particles, which change their direction in galactic magnetic fields; moreover, as they hardly interact they can be easily observed from across the galaxy. Gamma rays therefore offer a perfect means for reconstructing the distribution or halo profile of dark matter though observations in different sky directions.
Of course this assumes that gamma rays from dark-matter annihilation can be differentiated from the background, but this is indeed possible, since the spectral shapes are very different, as can be understood as follows. WIMPs have almost no kinetic energy, so after their annihilation into quark pairs the WIMP mass is converted into the energy of the quarks. The gamma rays produced in the fragmentation of such mono-energetic quarks have been well studied at CERN’s Large Electron Positron collider; they originate mainly from the decay of the copiously produced π0 mesons. The background, on the other hand, originates predominantly from the decay of π0 mesons produced by cosmic rays (mainly protons) scattering inelastically on the gas of the galactic disc, and so corresponds to the spectrum of gamma rays produced in fixed-target experiments with proton-proton collisions. In this case the gamma-ray spectrum can be calculated from the known cosmic-ray spectrum.
Clearly the steep power-law spectrum of cosmic rays will yield a spectrum of gamma rays that differs from that of the mono-energetic quarks produced in dark-matter annihilation. These different shapes can therefore be fitted to the data with free normalization factors, which then determine the relative contributions from dark-matter annihilation and background. Fitting the shapes has the advantage that the amount of background is determined from the data itself in each sky direction, so there is no need to rely on complicated galactic propagation models to obtain absolute background fluxes.
So what can be seen in the gamma-ray sky? A very detailed gamma-ray distribution over the whole sky was obtained by the Energetic Gamma Ray Emission Telescope (EGRET) on NASA’s Compton Gamma Ray Observatory, which collected data from 1991 to 2000. The EGRET telescope was carefully calibrated at SLAC in a quasi-monochromatic photon beam in the energy range 0.02 to 10 GeV. In 1997 the EGRET collaboration published their findings on a diffuse component of the gamma rays that cannot be described by the background: they observed an excess as large as a factor of two above the background for gamma-ray energies above 1 GeV. Recently, at the University of Karlsruhe, we have shown that this apparent excess traces the distribution of dark matter, since knowing the distribution of both the visible and dark matter allows us to reconstruct the rotation curve of our galaxy, especially its peculiar non-flat shape, which can be explained by the EGRET excess.
Mapping the flux
Figure 1 shows the excess for the flux from the galactic centre. The curve through the data points corresponds to the two-parameter fit, where the parameters are the normalization factors for the two known spectral shapes of signal and background, as discussed above; the red and yellow areas indicate the contributions from the dark-matter annihilation signal and the background, respectively. The WIMP mass was taken to be 60 GeV, which gives an excellent fit, although WIMP masses between 50 and 100 GeV are allowed, if extremes of the background shapes are allowed. The fit was repeated for 180 independent sky directions. In every direction the excess was observed and in every direction an excellent fit could be obtained for a WIMP mass of 60 GeV, if the contribution from the extragalactic background was also taken into account towards the galactic poles.
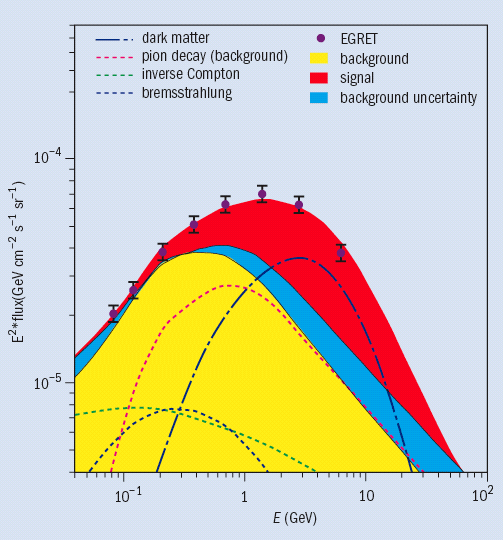
Such a detailed mapping of the flux of dark-matter annihilation in the sky allows a reconstruction of the distribution of dark matter in our galaxy. The result is surprising: it yields a pseudo-isothermal profile, as observed from the rotation curves in many galaxies, but with a substructure in the galactic plane in the form of doughnut-shaped rings at radii of 4 and 14 kpc. The position of our solar system at a distance of 8 kpc from the centre is located between this inner and outer ring. The enhanced gamma radiation at 14 kpc was also discussed in the original paper by Hunter et al. in 1997 and called the “cosmic enhancement factor”.
The ring structures in the dark-matter halo are expected to have a significant influence. A star inside the outer ring will feel an inward gravitational force from the galactic centre and an outward force from the outer ring, so the total gravitational force is reduced. This means that fast stars will go out of orbit inside the outer ring, thus causing a minimum in the rotation curve for radii within the outer ring. Outside the ring the gravitational forces from the centre and the ring add together, thus providing a maximum in the rotation curve. These effects are indeed observed, as shown in figure 2, indicating that the EGRET excess really does trace the dark matter in our galaxy.
The origin of these substructures in the dark-matter distribution is thought to be the hierarchical clustering of dark matter into galaxies: small clumps of dark matter grow from the quantum fluctuations appearing after inflation in the early universe and these clumps combine to form galaxies. That the outer ring originates from the infall of a dwarf galaxy is supported by the fact that hundreds of millions of old, mostly burned-out stars have recently been discovered in this region (Newberg et al. 2002, Ibata et al. 2003 and Crane et al. 2003). The small velocity dispersion and large-scale height perpendicular to the galactic disc of these stars proves that they cannot be part of the disc.

The position and shape of the inner ring coincides with a ring of molecular hydrogen. Molecules form from atomic hydrogen in the presence of dust or heavy nuclei, so a ring of neutral hydrogen suggests an attractive gravitational potential well in which the dust can settle. The significant contribution of the inner ring to the rotation curve is also indicated in figure 2.
The perfect WIMP
The conclusion that the EGRET excess traces dark matter makes no assumption about the nature of the dark matter, except that its annihilation produces hard gamma rays consistent with the fragmentation of mono-energetic quarks between 50 and 100 GeV. Supersymmetry, which presupposes a symmetry between particles with even spin (bosons) and odd spin (fermions), provides a good WIMP candidate. This symmetry requires a doubling of the particle species in the Standard Model: each boson obtains a fermion as superpartner and vice versa. These superpartners are still to be found, but the lightest is expected to be stable, neutral, massive and barely interacting with normal matter, i.e. it is the perfect WIMP.
Although the present data cannot prove the supersymmetric nature of dark matter, it is intriguing that the WIMP mass and WIMP annihilation cross-section (which can be calculated from the present WIMP density) are perfectly compatible with supersymmetry, including all constraints from electroweak precision experiments and limits from direct searches for Higgs bosons and supersymmetric particles, at least if the spin-0 superpartners are in the tera-electron-volt range. Figure 3 shows the allowed range of masses for spin-0 and spin-½ superpartners, assuming mass unification at the grand unification scale, i.e. common masses m0 (m½) for the spin-0 (½) supersymmetric particles.
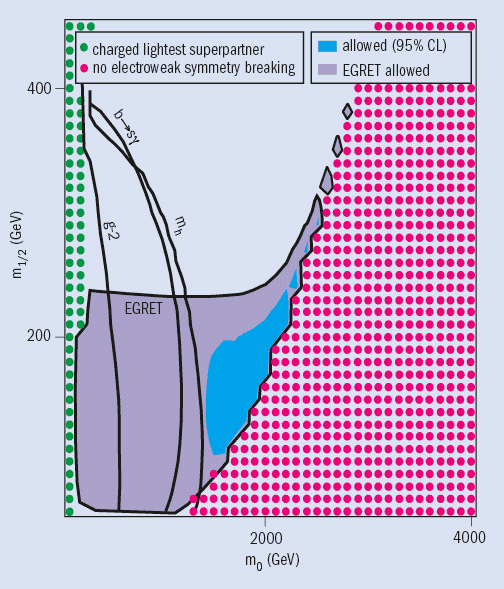
The allowed region in figure 3 is within reach of the Large Hadron Collider, so finding the predicted spectrum of light spin-½ and heavy spin-0 superpartners would prove the supersymmetric nature of the WIMP, especially if the lightest superpartner is stable and has the same mass as the WIMP mass deduced from the EGRET data. The lightest superpartner has properties akin to a spin-½ photon for the allowed region of figure 3, in which case the dark matter could be considered the supersymmetric partner of the cosmic microwave background, if supersymmetry is discovered. It is interesting to note that this region of parameter space yields perfect unification of the gauge couplings without any free parameters. In our first analysis in 1991, the scale of the supersymmetric masses had to be treated as a free parameter (Amaldi et al. 1991).
The statistical significance of the EGRET excess is at least 10 s and alternative models without dark matter do not yield good fits if all sky directions are considered. Furthermore, alternative models do not explain the peculiar shape of the rotation curve, or the occurrence of the hydrogen rings at 4 and 14 kpc and the high density of old stars at 14 kpc. Therefore, we conclude that the EGRET excess provides an intriguing hint that dark matter is not so dark, but is visible by flashes of typically 30-40 gamma rays for each annihilation.
Further reading
U Amaldi, W de Boer and H Fürstenau 1991 Phys. Lett. 260 447.
W de Boer, C Sander, V Zhukov, A V Gladyshev and D I Kazakov 2005 Astron. Astrophys. (in press) http://arxiv.org/abs/astro-ph/0508617.
J D Crane et al. 2003 ApJ 594 L119.
S D Hunter et al. 1997 ApJ 481 205.
R A Ibata et al. 2003 Mon. Not. Roy. Astron. Soc. 340 L21.
H J Newberg et al. 2002 ApJ 569 245.