Just like particle physics, cosmology has its own standard model. It is also powerful in prediction, and brings new mysteries and profound implications. The first was the realisation in 1917 that a homogeneous and isotropic universe must be expanding. This led Einstein to modify his general theory of relativity by introducing a cosmological constant (Λ) to counteract gravity and achieve a static universe – an act he labelled his greatest blunder when Edwin Hubble provided observational proof of the universe’s expansion in 1929. Sixty-nine years later, Saul Perlmutter, Adam Riess and Brian Schmidt went further. Their observations of Type Ia supernovae (SN Ia) showed that the universe’s expansion was accelerating. Λ was revived as “dark energy”, now estimated to account for 68% of the total energy density of the universe.
On large scales the dominant motion of galaxies is the Hubble flow, the expansion of the fabric of space itself
The second dominant component of the model emerged not from theory but from 50 years of astrophysical sleuthing. From the “missing mass problem” in the Coma galaxy cluster in the 1930s to anomalous galaxy-rotation curves in the 1970s, evidence built up that additional gravitational heft was needed to explain the formation of the large-scale structure of galaxies that we observe today. The 1980s therefore saw the proposal of cold dark matter (CDM), now estimated to account for 27% of the energy density of the universe, and actively sought by diverse experiments across the globe and in space.
Dark energy and CDM supplement the remaining 5% of normal matter to form the ΛCDM model. ΛCDM is a remarkable six-parameter framework that models 13.8 billion years of cosmic evolution from quantum fluctuations during an initial phase of “inflation” – a hypothesised expansion of the universe by 26 to 30 orders of magnitude in roughly 10–36 seconds at the beginning of time. ΛCDM successfully models cosmic microwave background (CMB) anisotropies, the large-scale structure of the universe, and the redshifts and distances of SN Ia. It achieves this despite big open questions: the nature of dark matter, the nature of dark energy and the mechanism for inflation.
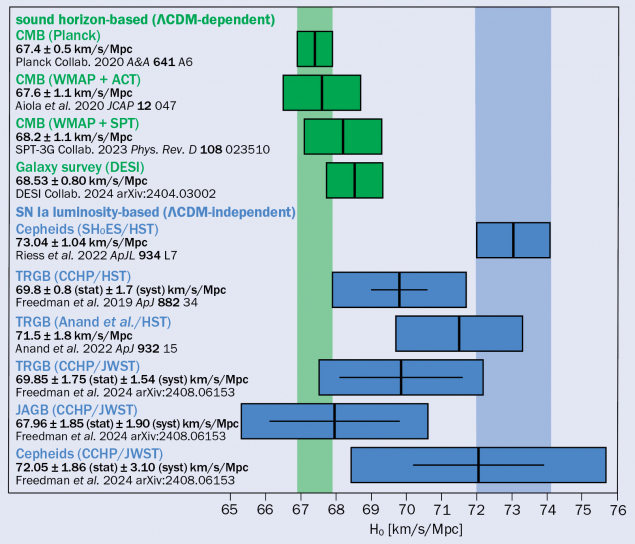
Cosmologists are eager to guide beyond-ΛCDM model-building efforts by testing its end-to-end predictions, and the model now seems to be failing the most important: predicting the expansion rate of the universe.
One of the main predictions of ΛCDM is the average energy density of the universe today. This determines its current expansion rate, otherwise known as the Hubble constant (H0). The most precise ΛCDM prediction comes from a fit to CMB data from ESA’s Planck satellite (operational 2009 to 2013), which yields H0 = 67.4 ± 0.5 km/s/Mpc. This can be tested against direct measurements in our local universe, revealing a surprising discrepancy (see “The Hubble tension” figure).
At sufficiently large distances, the dominant motion of galaxies is the Hubble flow – the expansion of the fabric of space itself. Directly measuring the expansion rate of the universe calls for fitting the increase in the recession velocity of galaxies deep within the Hubble flow as a function of distance. The gradient is H0.
Receding supernovae
While high-precision spectroscopy allows recession velocity to be precisely measured using the redshifts (z) of atomic spectra, it is more difficult to measure the distance to astrophysical objects. Geometrical methods such as parallax are imprecise at large distances, but “standard candles” with somewhat predictable luminosities such as cepheids and SN Ia allow distance to be inferred using the inverse square-law. Cepheids are pulsating post-main-sequence stars whose radius and observed luminosity oscillate over a period of one to 100 days, driven by the ionisation and recombination of helium in their outer layers, which increases opacity and traps heat; their period increases with their true luminosity. Before going supernova, SN Ia were white dwarf stars in binary systems; when the white dwarf accretes enough mass from its companion star, runaway carbon fusion produces a nearly standardised peak luminosity for a period of one to two weeks. Only SN Ia are deep enough in the Hubble flow to allow precise measurements of H0. When cepheids are observable in the same galaxies, they can be used to calibrate them.
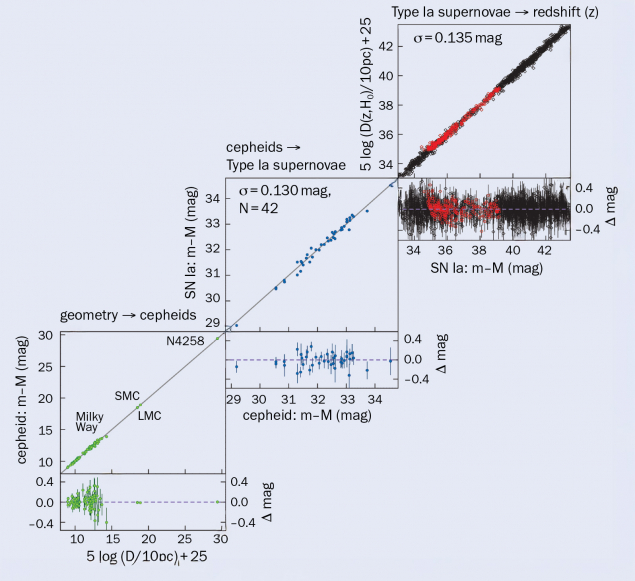
At present, the main driver of the Hubble tension is a 2022 measurement of H0 by the SH0ES (Supernova H0 for the Equation of State) team led by Adam Riess. As the SN Ia luminosity is not known from first principles, SH0ES built a “distance ladder” to calibrate the luminosity of 42 SN Ia within 37 host galaxies. The SN Ia are calibrated against intermediate-distance cepheids, and the cepheids are calibrated against four nearby “geometric anchors” whose distance is known through a geometric method (see “Distance ladder” figure). The geometric anchors are: Milky Way parallaxes from ESA’s Gaia mission; detached eclipsing binaries in the large and small magellanic clouds (LMC and SMC); and the “megamaser” galaxy host NGC4258, where water molecules in the accretion disk of a supermassive black hole emit Doppler-shifting microwave maser photons.
The great strength of the SH0ES programme is its use of NASA and ESA’s Hubble Space Telescope (HST, 1990–) at all three rungs of the distance ladder, bypassing the need for cross-calibration between instruments. SN Ia can be calibrated out to 40 Mpc. As a result, in 2022 SH0ES used measurements of 300 or so high-z SN Ia deep within the Hubble flow to measure H0 = 73.04 ± 1.04 km/s/Mpc. This is in more than 5σ tension with Planck’s ΛCDM prediction of 67.4 ± 0.5 km/s/Mpc.
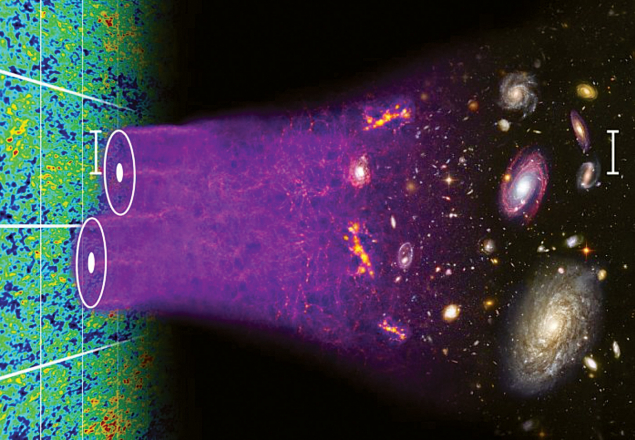
The sound horizon
The value of H0 obtained from fitting Planck CMB data has been shown to be robust in two key ways.
First, Planck data can be bypassed by combining CMB data from NASA’s WMAP probe (2001–2010) with observations by ground-based telescopes. WMAP in combination with the Atacama Cosmology Telescope (ACT, 2007–2022) yields H0 = 67.6 ± 1.1 km/s/Mpc. WMAP in combination with the South Pole Telescope (SPT, 2007–) yields H0 = 68.2 ± 1.1 km/s/Mpc. Second, and more intriguingly, CMB data can be bypassed altogether.
In the early universe, Compton scattering between photons and electrons was so prevalent that the universe behaved as a plasma. Quantum fluctuations from the era of inflation propagated like sound waves until the era of recombination, when the universe had cooled sufficiently for CMB photons to escape the plasma when protons and electrons combined to form neutral atoms. This propagation of inflationary perturbations left a characteristic scale known as the sound horizon in both the acoustic peaks of the CMB and in “baryon acoustic oscillations” (BAOs) seen in the large-scale structure of galaxy surveys (see “Baryon acoustic oscillation” figure). The sound horizon is the distance travelled by sound waves in the primordial plasma.
While the SH0ES measurement relies on standard candles, ΛCDM predictions rely instead on using the sound horizon as a “standard ruler” against which to compare the apparent size of BAOs at different redshifts, and thereby deduce the expansion rate of the universe. Under ΛCDM, the only two free parameters entering the computation of the sound horizon are the baryon density and the dark-matter density. Planck evaluates both by studying the CMB, but they can be obtained independently of the CMB by combining BAO measurements of the dark-matter density with Big Bang nucleosynthesis (BBN) measurements of the baryon density (see “Sound horizon” figure). The latest measurement by the Dark Energy Spectroscopic Instrument in Arizona (DESI, 2021–) yields H0 = 68.53 ± 0.80 km/s/Mpc, in 3.4σ tension with SH0ES and fully independent of Planck.
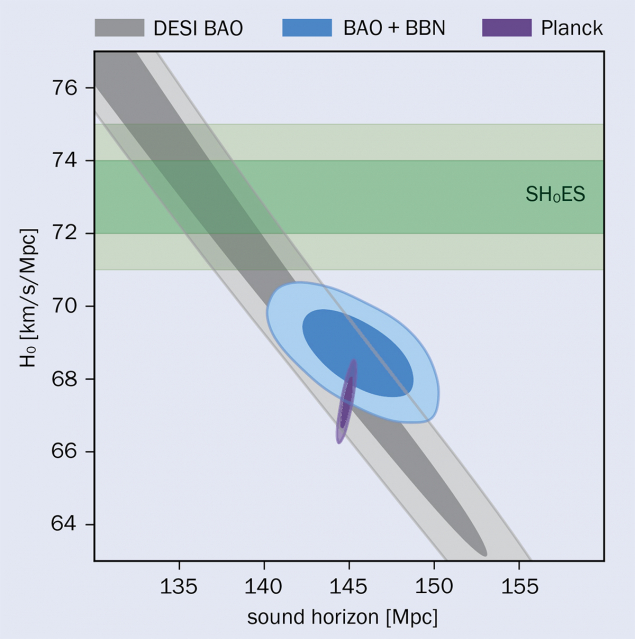
The next few years will be crucial for understanding the Hubble tension, and may decide the fate of the ΛCDM model. ACT, SPT and the Simons Observatory in Chile (2024–) will release new CMB data. DESI, the Euclid space telescope (2023–) and the forthcoming LSST wide-field optical survey in Chile will release new galaxy surveys. “Standard siren” measurements from gravitational waves with electromagnetic counterparts may also contribute to the debate, although the original excitement has dampened with a lack of new events after GW170817. More accurate measurements of the age of the oldest objects may also provide an important new test. If H0 increases, the age of the universe decreases, and the SH0ES measurement favours less than 13.1 billion years at 2σ significance.
The SH0ES measurement is also being checked directly. A key approach is to test the three-step calibration by seeking alternative intermediate standard candles besides cepheids. One candidate is the peak-luminosity “tip” of the red giant branch (TRGB) caused by the sudden start of helium fusion in low-mass stars. The TRGB is bright enough to be seen in distant galaxies that host SN Ia, though at distances smaller than that of cepheids.
Settling the debate
In 2019 the Carnegie–Chicago Hubble Program (CCHP) led by Wendy Freedman and Barry Madore calibrated SN Ia using the TRGB within the LMC and NGC4258 to determine H0 = 69.8 ± 0.8 (stat) ± 1.7 (syst). An independent reanalysis including authors from the SH0ES collaboration later reported H0 = 71.5 ± 1.8 (stat + syst) km/s/Mpc. The difference in the results suggests that updated measurements with the James Webb Space Telescope (JWST) may settle the debate.
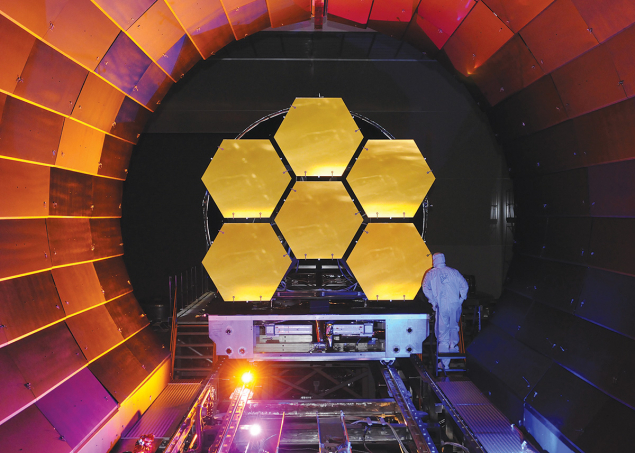
Launched into space on 25 December 2021, JWST is perfectly adapted to improve measurements of the expansion rate of the universe thanks to its improved capabilities in the near infrared band, where the impact of dust is reduced (see “Improved resolution” figure). Its four-times-better spatial resolution has already been used to re-observe a subsample of the 37 hosts galaxies home to the 42 SN Ia studied by SH0ES and the geometric anchor NGC4258.
So far, all observations suggest good agreement with the previous observations by HST. SH0ES used JWST observations to obtain up to a factor 2.5 reduction in the dispersion of the period-luminosity relation for cepheids with no indication of a bias in HST measurements. Most importantly, they were able to exclude the confusion of cepheids with other stars as being responsible for the Hubble tension at 8σ significance.
Meanwhile, the CCHP team provided new measurements based on three distance indicators: cepheids, the TRGB and a new “population based” method using the J-region of the asymptotic giant branch (JAGB) of carbon-rich stars, for which the magnitude of the mode of the luminosity function can serve as a distance indicator (see the last three rows of “The Hubble tension” figure).
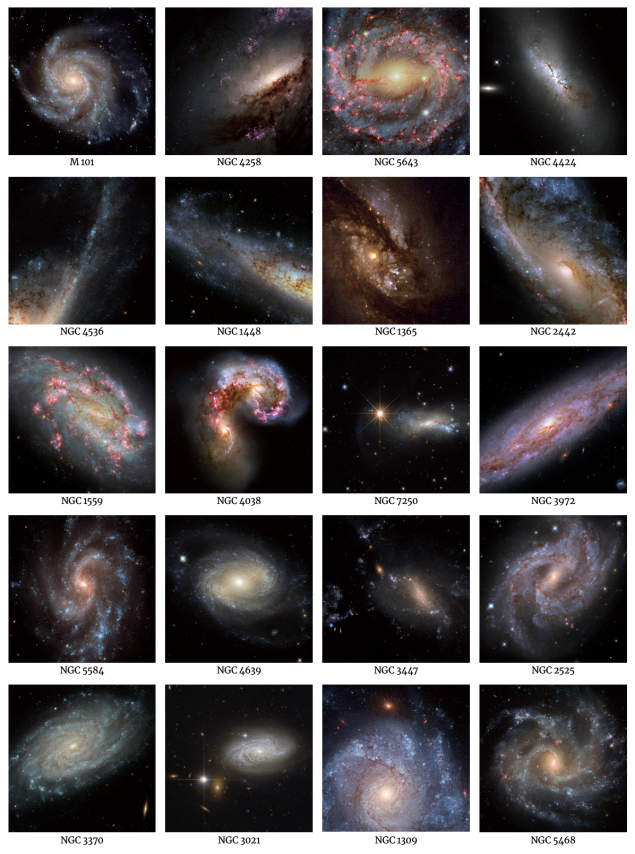
The new CCHP results suggest that cepheids may show a bias compared to JAGB and TRGB, though this conclusion was rapidly challenged by SH0ES, who identified a missing source of uncertainty and argued that the size of the sample of SN Ia within hosts with primary distance indicators is too small to provide competitive constraints: they claim that sample variations of order 2.5 km/s/Mpc could explain why the JAGB and TRGB yield a lower value. Agreement may be reached when JWST has observed a larger sample of galaxies – across both teams, 19 of the 37 calibrated by SH0ES have been remeasured so far, plus the geometric anchor NGC 5468 (see “The usual suspects” figure).
At this stage, no single systematic error seems likely to fully explain the Hubble tension, and the problem is more severe than it appears. When calibrated, SN Ia and BAOs constrain not only H0, but the entire redshift range out to z ~ 1. This imposes strong constraints on any new physics introduced in the late universe. For example, recent DESI results suggest that the dynamics of dark energy at late times may not be exactly that of a cosmological constant, but the behaviour needed to reconcile Planck and SH0ES is strongly excluded.
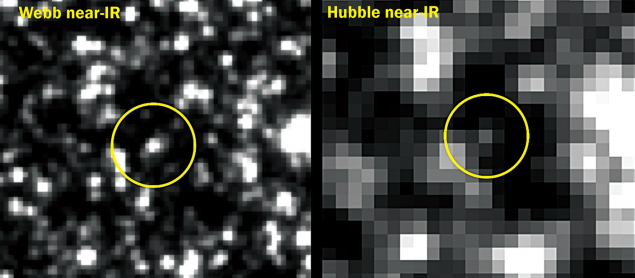
Rather than focusing on the value of the expansion rate, most proposals now focus on altering the calibration of either SN Ia or BAOs. For example, an unknown systematic error could alter the luminosity of SN Ia in our local vicinity, but we have no indication that their magnitude changes with redshift, and this solution appears to be very constrained.
The most promising solution appears to be that some new physics may have altered the value of the sound horizon in the early universe. As the sound horizon is used to calibrate both the CMB and BAOs, reducing it by 10 Mpc could match the value of H0 favoured by SH0ES (see “Sound horizon” figure). This can be achieved either by increasing the redshift of recombination or the energy density in the pre-recombination universe, giving the sound waves less time to propagate.
The best motivated models invoke additional relativistic species in the early universe such as a sterile neutrino or a new type of “dark radiation”. Another intriguing possibility is that dark energy played a role in the pre-recombination universe, boosting the expansion rate at just the right time. The wide variety and high precision of the data make it hard to find a simple mechanism that is not strongly constrained or finely tuned, but existing models have some of the right features. Future data will be decisive in testing them.