With their ultrashort wavelengths, X-rays are excellent probes of finestructure. An international team at the DESY laboratory has developed a technique for attaining wavelengths of less than 100 nm.
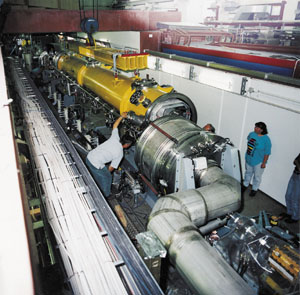
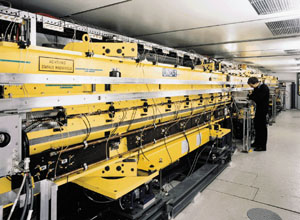
On 22 February an international team working at the superconducting TESLA Test Facility (TTF) at DESY, Hamburg, set a new record for the shortest wavelength of radiation ever achieved with a free electron laser (FEL). The collaboration, involving around 140 scientists from 38 institutes in 9 countries, succeeded in generating ultraviolet radiation with a wavelength of 109 nm. The previous best using this type of self-amplified spontaneous emission (SASE) FEL was 530 nm (by a group at Argonne). Within a few weeks the group pushed the wavelength down to 80 nm and tuned the FEL to various wavelengths up to 180 nm, thus demonstrating for the first time the free wavelength tunability of SASE FELs over a large range.
To achieve this decisive step towards a new range of laser wavelengths, the TESLA team used the electron beam from the superconducting test linac of the TTF, set up for the development and testing of new superconducting niobium cavities for DESY’s planned 33 km TESLA electron-positron linear collider.
Having demonstrated that SASE is working at such small wavelengths, the collaboration is now extending the short TTF into a 300 m device that could operate in the soft X-ray range around 6 nm. This user facility should be available for experiments by 2003.
The ultimate goal is to produce X-rays with a wavelength of 0.1 nm. Since these short wavelengths require an electron beam of much higher energy, this X-ray laser facility is planned to be included as an integral part of the proposed TESLA linear collider.
Synchrotron radiation
Since its discovery in the mid-1940s, synchrotron radiation has evolved into an invaluable tool for experiments in a range of fields – from surface physics, materials sciences and chemistry, to geophysics, molecular biology and medicine. Nowadays, third -generation synchrotron radiation sources are producing nearly every wavelength from infrared to hard X-rays. However, one thing has so far remained a dream: beams of coherent high-intensity X-rays – a high-intensity X-ray laser.
How to reach this “ultimate” X-ray source has been the subject of many effort both theoretically and experimentally in the last 20 years. During the past 6-8 years a consensus has developed that fourth-generation sources, implying a higher degree of coherence, higher power, brilliance and ultrashort pulses, possibly at very short wavelengths, such as the hard X-ray region, would probably involve a linear accelerator driving a FEL.
This shift from storage rings to linear devices is because the quality of the electron beam – short bunch lengths and small beam emittance – is limited at storage rings but is crucially important for the FEL process. Especially when aiming at X-ray wavelengths with a SASE FEL, the limitations of storage rings become noticeable.
Free electron lasers
The main ingredients of a FEL are a high-energy electron beam with very high brightness and a periodic transverse magnetic field, such as that produced by an undulator magnet. As the electron bunches zigzag through the magnetic field, they emit synchrotron radiation around their direction of motion. For small undulations the radiation is quasimonochromatic. For every undulator period the radiation phase moves ahead of the electrons by a distance equal to this specific resonant wavelength, keeping each electron in phase with the radiation field.
Depending on the relative phase between radiation and electron oscillation, electrons experience either a retardation or an acceleration with respect to the mean electron velocity.
If the electron beam is of sufficient quality and the undulator long enough, the longitudinal density of the electron bunch becomes modulated, with “microbunching” at the resonant wavelength. This electron density modulation reduces phase cancellation in the emission process, increasing the intensity of the emitted light. This light interacts again with the electron beam and again enhances the bunch density modulation, thereby further increasing the intensity. The net result is an exponential increase in radiated power – ultimately about six orders of magnitude more brilliant than conventional undulator radiation.
No mirrors
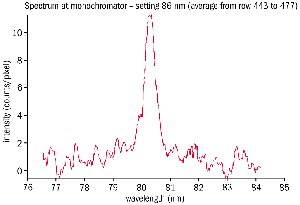
Like conventional lasers, most present FELs use an optical cavity formed by mirrors to store the light from many successive electron bunches. Many of these FELs work in the infrared range, and some even reach ultraviolet wavelengths. However, extending them towards the X-ray regime is difficult, owing to the lack of good reflecting surfaces at wavelengths below 150 nm.
An alternative path to shorter wavelengths was found with the development of SASE FELs. These achieve lasing in the single pass of a high-brightness electron bunch through a very long undulator by SASE process, without any mirrors.
The concept of SASE FELs was introduced in the early 1980s (Kondratenko and Saldin 1980) and further explored in 1984 (Bonifacio et al.1984), soon leading to first experimental tests (Scharlemann et al.1986). During 1997-8 a Los Alamos/UCLA experiment at Los Alamos (Hogan et al.1998) produced a gain of 3 x 105 for the first time and established the proof-of-principle of SASE theory at a wavelength of 12 mm. Recently, SASE at 530 nm was demonstrated at Argonne (Milton et al.submitted).
Testbed
The TTF was set up at DESY in 1993, with major contributions from the US, Italy and France, to provide a testbed for the TESLA linear collider project, especially the superconducting niobium cavities for particle acceleration. In 1994 work began on the test accelerator to extend it into a 300 m FEL comprising all of the basic elements that will subsequently be employed in full-size TESLA X-ray lasers.
In a first phase, now brought to laser operation, the TTF was equipped with a 15 m undulator, a bunch compressor (reducing the bunch length, thus increasing the bunch peak current) and a radiofrequency photocathode electron gun.
There are essentially two technical challenges to be met by an X-ray FEL. First, it is crucial to generate and accelerate a low emittance and high-peak-current electron beam. This can be achieved using a high-brightness radiofrequency photocathode gun as an electron source. The electron gun currently used at the TTF-FEL is a joint contribution of Fermilab, INFN/Milan, Rochester, the Max Born Institute in Berlin and DESY.
It has meanwhile demonstrated that such a particle source can drive a facility 24 h a day for weeks and even months. Because the radiofrequency gun performance is so critical for further development, DESY is building up a standalone gun test facility at its institute in Zeuthen near Berlin.
The peak current inside the bunches produced by the low-emittance gun is still not high enough to reach laser saturation within an undulator of reasonable length. The solution is to compress the bunches longitudinally to increase the peak current. This can be achieved using a “bunch compressor chicane” – a sequence of deflecting magnets.
The principle is not new, but aiming at a few kiloamperes of peak current means achieving bunch lengths of less than 0.1 mm, which is a challenge. Accelerating the beam off the crest of the radiofrequency waveform in the linac creates an energy-phase correlation that can be used to shorten the bunch. When passing the chicane, electrons with different momenta travel different path lengths. The TTF-FEL currently uses a bunch compressor at 140 MeV, which compresses the bunch length below 0.5 mm rms.
The second important technical challenge is to keep the electron beam (focused to a transverse beam size of about 0.1 mm) in essentially complete overlap with the photon beam as it passes through the undulator. This sets new standards for undulator alignment procedures and beam orbit control.
Interleaved operation
Combining the machine expertise at a high-energy physics facility with operation of a radiation source continues a long and fruitful tradition at DESY. Technically, both X-ray SASE FELs and linear colliders depend fundamentally on the generation of low-emittance, short electron bunches and on accelerating long bunch trains without loss of this quality. This is best achieved with a superconducting linac, combining high accelerating gradients and low wakefield effects with long bunch trains at high duty cycle, owing to low power losses.
For power cost reasons, a superconducting linear collider has a radiofrequency-on-time fraction of only 1%. Consequently there is room for further radiofrequency pulses to accelerate an interleaved electron beam for FEL operation. In this way the most expensive component of an X-ray laser – the linac – is shared with the high-energy physics community.
All TTF findings are consistent with existing models for SASE FELs. So far, a laser gain of more than 1000 has been observed, while laser saturation is expected well beyond 106. Thus the next steps will be focused on achieving even higher laser gain by improving orbit control and electron beam quality. Operation with long trains of several thousand electron bunches will also be tested.
Having accomplished the proof-of-principle experiment (Andruszkow et al.),the TESLA collaboration will then upgrade the superconducting linac to 1000 MeV (Åberg et al.1995), bringing the FEL wavelength down to 6 nm. The new user facility should be ready for experiments by 2003. As for the TESLA Linear Collider with Integrated X-ray Lasers, a conceptual design was published in 1997 (Brinkman et al.1997) and a Technical Design Report, including schedule and costs, will be presented in 2001 for evaluation by the German Science Council (Wissenschaftsrat), the German Federal Government’s scientific advisory board. As a first step towards formal planning permission, an agreement was signed in 1998 by the relevant German federal states.
Further reading
A M Kondratenko and E L Saldin 1980 Generation of coherent radiation by a relativistic electron beam in an ondulator Part. Accelerators10 207-216.
R Bonifacio, C Pellegrini and L Naducci 1984 Collective instabilities and high gain regime in a free electron laser Opt. Commun.50(6) 373-378.
E T Scharlemann et al.1986 Comparison of the Livermore microwave FEL results at ELF with 2D numerical simulations Proceedings of the Seventh FEL Conference (FEL86)A250 150-158.
M J Hogan et al.1998 Measurements of gain larger than 105 at 12 mm in a self-amplified spontaneous-emission free-electron laser Phys. Rev. Lett.81 4867-4870.
S V Milton et al.submitted Observation of self-amplified spontaneous emission and exponential growth at 530 nm.
J Andruszkow et al.First observation of self-amplified spontaneous emission in a free-electron laser at 109 nm wavelength, DESY 00-066.
T. Åberg et al.1995 A VUV FEL at the TESLA test facility at DESY, Conceptual Design Report DESY Print TESLA-FEL95-03.
R Brinkmann, G Materlik, J Rossbach and A Wagner (eds) 1997 Conceptual Design of a 500 GeV e+e– Linear Collider with Integrated X-ray Laser Facility(DESY 1997-048 and ECFA 1997-182).