The unknown origin of the matter–antimatter asymmetry observed in the universe looms large in the scientific imagination. Boris Kayser explains how neutrino physicists are now closing in on a crucial piece of evidence in this most convoluted of detective stories.
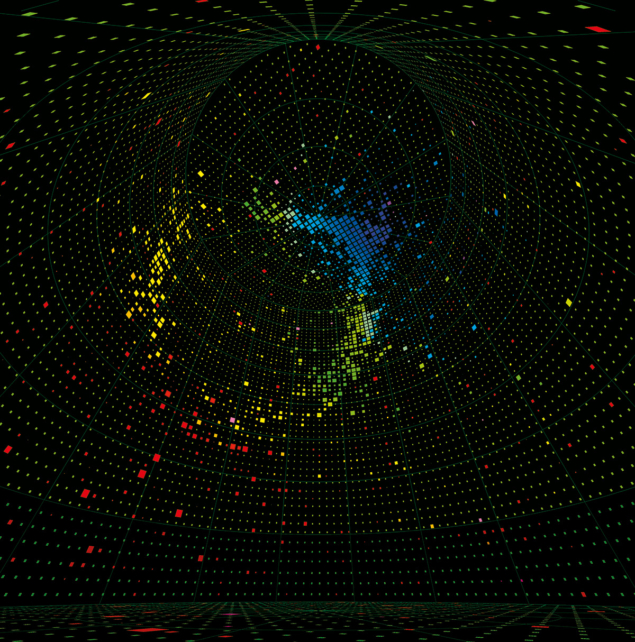
Luckily for us, there is presently almost no antimatter in the universe. This makes it possible for us – made of matter – to live without being annihilated in matter–antimatter encounters. However, cosmology tells us that just after the cosmic Big Bang, the universe contained equal amounts of matter and antimatter. Obviously, for the universe to have evolved from that early state to the present one, which contains quite unequal amounts of matter and antimatter, the two must behave differently. This implies that the symmetry CP (charge conjugation × parity) must be violated. That is, there must be physical systems whose behaviour changes if we replace every particle by its antiparticle, and interchange left and right.
In 1964, Cronin, Fitch and colleagues discovered that CP is indeed violated, in the decays of neutral kaons to pions – a phenomenon that later became understood in terms of the behaviour of quarks. By now, we have observed quark CP violation in the strange sector, the beauty sector and most recently in the charm sector (CERN Courier May/June 2019 p7). The observations of CP violation in B (beauty) meson decays have been particularly illuminating. Everything we know about quark CP violation is consistent with the hypothesis that this violation arises from a single complex phase in the quark mixing matrix. This matrix gives the amplitude for any particular negatively-charged quark, whether down, strange or bottom, to convert via a weak interaction into any particular positively-charged quark, be it up, charm or top. Just two parameters in the quark mixing matrix, ρ and η, whose relative size determines the complex phase, account very successfully for numerous quark phenomena, including both CP-violating ones and others. This is impressively demonstrated by a plot of all the experimental constraints on these two parameters (figure 1). All the constraints intersect at a common point.
Of course, precisely which (ρ, η) point is consistent with all the data is not important. Lincoln Wolfenstein, who created the quark-mixing-matrix parametrisation that includes ρ and η, was known to say: “Look, I invented ρ and η, and I don’t care what their values are, so why should you?”

Having observed CP violation among quarks in numerous laboratory experiments of today, we might be tempted to think that we understand how CP violation in the early universe could have changed the world from one with equal quantities of matter and antimatter to one in which matter dominates very heavily over antimatter. However, scenarios that tie early-universe CP violation to that seen among the quarks today, and do not add new physics to the Standard Model of the elementary particles, yield too small a present-day matter–antimatter asymmetry. This leads one to wonder whether early-universe CP violation involving leptons, rather than quarks, might have led to the present dominance of matter over antimatter. This possibility is envisaged by leptogenesis, a scenario in which heavy neutral leptons that were their own antiparticles lived briefly in the early universe, but then underwent CP-asymmetric decays, creating a world with unequal numbers of particles and antiparticles. Such heavy neutral leptons are predicted by “see-saw” models, which explain the extreme lightness of the known neutrinos in terms of the extreme heaviness of the postulated heavy neutral leptons. Leptogenesis can successfully account for the observed size of the present matter–antimatter asymmetry.
Deniable plausibility
In the straightforward version of this picture, the heavy neutral leptons are too massive to be observable at the LHC or any foreseen collider. However, since leptogenesis requires leptonic CP violation, observing this violation in the behaviour of the currently observed leptons would make it more plausible that leptogenesis was indeed the mechanism through which the present matter–antimatter asymmetry of the universe arose. Needless to say, observing leptonic CP violation would also reveal that the breaking of CP symmetry, which before 1964 one might have imagined to be an unbroken, fundamental symmetry of nature, is not something special to the quarks, but is participated in by all the constituents of matter.

To find out if leptons violate CP, we are searching for what is traditionally described as a difference between the behaviour of neutrinos and that of antineutrinos. This description is fine if neutrinos are Dirac particles – that is, particles that are distinct from their antiparticles. However, many theorists strongly suspect that neutrinos are actually Majorana particles – that is, particles that are identical to their antiparticles. In that case, the traditional description of the search for leptonic CP violation is clearly inapplicable, since then the neutrinos and the antineutrinos are the same objects. However, the actual experimental approach that is being pursued is a perfectly valid probe of leptonic CP violation regardless of whether neutrinos are of Dirac or of Majorana character. In fact, this approach is completely insensitive to which of these two possibilities nature has chosen.
Through a glass darkly
The pursuit of leptonic CP violation is based on comparing the rates for two CP mirror-image processes (figure 2). In process A, the initial state is a π+ and an undisturbed detector. The final state consists of a μ+, an e–, and a nucleus in the detector that has been struck by an intermediate-state neutrino beam particle that travelled a long distance from its source to the detector. Since the neutrino was born together with a muon, but produced an electron in the detector, and the probability for this to have happened oscillates as a function of the distance the neutrino travels divided by its energy, the process is commonly referred to as muon–neutrino to electron–neutrino oscillation.
Leptogenesis can account for the matter–antimatter asymmetry
In process B, the initial and final states are the same as in process A, but with every particle replaced by its antiparticle. In addition, owing to the character of the weak interactions, the helicity (the projection of the spin along the momentum) of every fermion is reversed, so that left and right are interchanged. Thus, regardless of whether neutrinos are identical to their antiparticles, processes A and B are CP mirror images, so if their rates are unequal, CP invariance is violated. Moreover, since the probability of a neutrino oscillation involves the weak interactions of leptons, but not those of quarks, this violation of CP invariance must come from the weak interactions of leptons.
Of course, we cannot employ an anti-detector in process B in practice. However, the experiment can legitimately use the same detector in both processes. To do that, it must take into account the difference between the cross sections for the beam particles in processes A and B to interact in this detector. Once that is done, the comparison of the rates for processes A and B remains a valid probe of CP non-invariance.
The matrix reloaded
Just as quark CP violation arises from a complex phase in the quark mixing matrix, so leptonic CP violation in neutrino oscillation can arise from a complex phase, δCP, in the leptonic mixing matrix, which is the leptonic analogue of the quark mixing matrix. However, if, as suggested by several short-baseline oscillation experiments, there exist not only the three well-established neutrinos, but also additional so-called “sterile” neutrinos that do not participate in Standard Model weak interactions, then the leptonic mixing matrix is larger than the quark one. As a result, while the quark mixing matrix is permitted to contain just one complex phase, its leptonic analogue may contain multiple complex phases that can contribute to CP violation in neutrino oscillations.
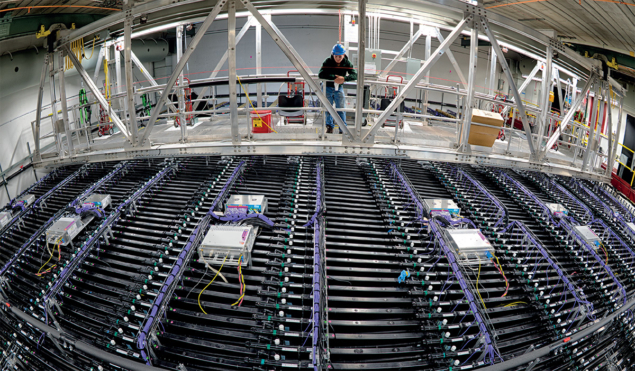
Leptonic CP violation is being sought by two current neutrino-oscillation experiments. The NOvA experiment in the US has reported results that are consistent with either the presence or absence of CP violation. The T2K experiment in Japan reports that the complete absence of CP violation is excluded at 95% confidence. Assuming that the leptonic mixing matrix is the same size as the quark one, so that it may contain only one complex phase relevant to neutrino oscillations, the T2K data show a preference for values of that phase, δCP, that correspond to near maximal CP violation. Of course, as Lincoln Wolfenstein would doubtless point out, the precise value of δCP is not important. What counts is the extremely interesting experimental finding that the behaviour of leptons may very well violate CP. In the future, the oscillation experiments Hyper-Kamiokande in Japan and DUNE in the US will probe leptonic CP violation with greater sensitivity, and should be capable of observing it even if it should prove to be fairly small (see Tuning in to neutrinos).
By searching for leptonic CP violation, we hope to find out whether the breaking of CP symmetry occurs among all the constituents of matter, including both the leptons and the quarks, or whether it is a feature that is special to the quarks. If leptonic CP violation should be definitively shown to exist, this violation might be related to the reason that the universe contains matter, but almost no antimatter, so that life is possible.
Further reading
The NOvA Collaboration 2019 Phys. Rev. Lett. 123 151803.
The T2K Collaboration 2020 Nature 580 339.