The Electron–Ion Collider (EIC) will help researchers to understand how visible matter emerges from fundamental quarks and gluons. As Jim Yeck, Ferdinand Willeke and Tom Ludlam explain, the engagement – at scale – of international partners and early-career scientists will be pivotal for successful delivery of this next-generation collider.

The international nuclear-physics community will be front-and-centre as a unique research facility called the Electron–Ion Collider (EIC) moves from concept to reality through the 2020s – the latest progression in the line of large-scale accelerator programmes designed to probe the fundamental forces and particles that underpin the structure of matter.
Decades of research in particle and nuclear physics have shown that protons and neutrons, once thought to be elementary, have a rich, dynamically complex internal structure of quarks, anti-quarks and gluons, the understanding of which is fundamental to the nature of matter as we experience it. By colliding high-energy beams of electrons with high-energy beams of protons and heavy ions, the EIC is designed to explore this hidden subatomic landscape with the resolving power to image its behaviour directly. Put another way: the EIC will provide the world’s most powerful microscope for studying the “glue” that binds the building blocks of matter.
Luminous performance
When the EIC comes online in the early 2030s, the facility will perform precision “nuclear femtography” by zeroing in on the substructure of quarks and gluons in a manner comparable to the seminal studies of the proton using electron–proton collisions at DESY’s HERA accelerator in Germany between 1992 and 2007 (see “Nuclear femtography to delve deep into nuclear matter” panel). However, the EIC will produce a luminosity (collision rate) 100 times greater than the highest achieved by HERA and, for the first time in such a collider, will provide spin-polarised beams of both protons and electrons, as well as high-energy collisions of electrons with heavy ions. All of which will require unprecedented performance in terms of the power, intensity and spatial precision of the colliding beams, with the EIC expected to provide not only transformational advances in nuclear science, but also transferable technology innovations to shape the next generation of particle accelerators and detectors.
The US Department of Energy (DOE) formally initiated the EIC project in December 2019 with the approval of a “mission need”. That was followed in June of this year with the next “critical decision” to proceed with funding for engineering and design prior to construction (with the estimated cost of the build about $2 billion). The new facility will be sited at Brookhaven National Laboratory (BNL) in Long Island, New York, utilising components and infrastructure from BNL’s Relativistic Heavy Ion Collider (RHIC), including the polarised proton and ion-beam capability and the 3.8 km underground tunnel. Construction will be carried out as a partnership between BNL and Thomas Jefferson National Accelerator Facility (JLab) in Newport News, Virginia, home of the Continuous Electron Beam Accelerator Facility (CEBAF), which has pioneered many of the enabling technologies needed for the EIC’s new electron rings.
Beyond the BNL–JLab partnership, the EIC is very much a global research endeavour. While the facility is not scheduled to become operational until early in the next decade, an international community of scientists is already hard at work within the EIC User Group. Formed in 2016, the group now has around 1300 members – representing 265 universities and laboratories from 35 countries – engaged collectively on detector R&D, design and simulation as well as initial planning for the EIC’s experimental programme.
A cutting-edge accelerator facility
Being the latest addition to the line of particle colliders, the EIC represents a fundamental link in the chain of continuous R&D, knowledge transfer and innovation underpinning all manner of accelerator-related technologies and applications – from advanced particle therapy systems for the treatment of cancer to ion implantation in semiconductor manufacturing.
The images “The EIC in outline” and “Going underground” show the planned layout of the EIC, where the primary beams circulate inside the existing RHIC tunnel to enable the collisions of high-energy (5–18 GeV) electrons (and possibly positrons) with high-energy ion beams of up to 275 GeV/nucleon. One thing is certain: the operating parameters of the EIC, with luminosities of up to 1034 cm–2 s–1 and up to 85% beam polarisation, will push the design of the facility beyond the limits set by previous accelerator projects in a number of core technology areas.

For starters, the EIC will require significant advances in the field of superconducting radiofrequency (SRF) systems operating under high current conditions, including control of higher-order modes, beam RF stability and crab cavities. A major challenge is the achievement of strong cooling of intense proton and light-ion beams to manage emittance growth owing to intrabeam scattering. Such a capability will require unprecedented control of low-energy electron-beam quality with the help of ultrasensitive and precise photon detection technologies – innovations that will likely yield transferable benefits for other areas of research reliant on electron-beam technology (e.g. free-electron lasers).
The EIC design for strong cooling of the ion beams specifies a superconducting energy-recovery linac with a virtual beam power of 15 MW, an order-of-magnitude increase versus existing machines. With this environmentally friendly new technology, the rapidly cycling beam of low-energy electrons (150 MeV) is accelerated within the linac and passes through a cooling channel where it co-propagates with the ions. The cooling electron beam is then returned to the linac, timed to see the decelerating phase of the RF field, and the beam power is thus recovered for the next accelerating cycle – i.e. beam power is literally recycled after each cooling pass.
The EIC will also require complex operating schemes. A case in point: fresh, highly polarised electron bunches will need to be frequently injected into the electron storage ring without disturbing the collision operation of previously injected bunches. Further complexity comes in maximising the luminosity and polarisation over a large range of centre-of-mass energies and for the entire spectrum of ion beams. With a control system that can monitor hundreds of beam parameters in real-time, and with hundreds of points where the guiding magnetic fields can be tuned on the fly, there is a vast array of “knobs-to-be-turned” to optimise overall performance. Inevitably, this is a facility that will benefit from the use of artificial intelligence and machine-learning technologies to maximise its scientific output.
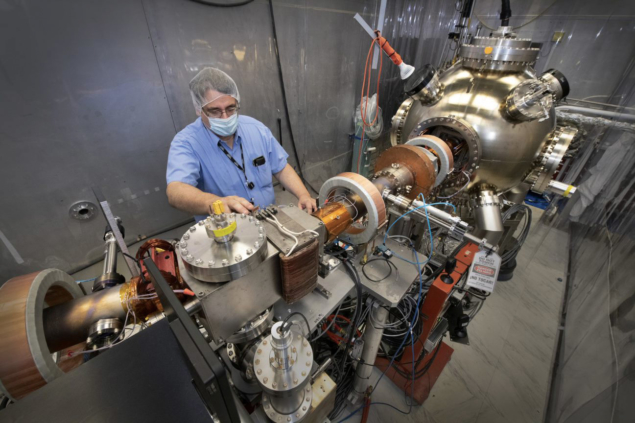
At the same time, the EIC and CERN’s High-Luminosity LHC user communities are working in tandem to realise more capable technologies for particle detection as well as innovative electronics for large-scale data read-out and processing. Exploiting advances in chip technology, with feature sizes as small as 65 nm, multipixel silicon sensors are in the works for charged-particle tracking, offering single-point spatial resolution better than 5 µm, very low mass and on-chip, individual-pixel readout. These R&D efforts open the way to compact arrays of thin solid-state detectors with broad angular coverage to replace large-volume gaseous detectors.
Coupled with leading-edge computing capabilities, such detectors will allow experiments to stream data continuously, rather than selecting small samples of collisions for readout. Taken together, these innovations will yield no shortage of downstream commercial opportunities, feeding into next-generation medical imaging systems, for example, as well as enhancing industrial R&D capacity at synchrotron light-source facilities.
The BNL–JLab partnership
As the lead project partners, BNL and JLab have a deep and long-standing interest in the EIC programme and its wider scientific mission. In 2019, BNL and JLab each submitted their own preconceptual designs to DOE for a future high-energy and high-luminosity polarised EIC based around existing accelerator infrastructure and facilities. In January 2020, DOE subsequently selected BNL as the preferred site for the EIC, after which the two labs immediately committed to a full partnership between their respective teams (and other collaborators) in the construction and operation of the facility.
Nuclear femtography to delve deep into nuclear matter
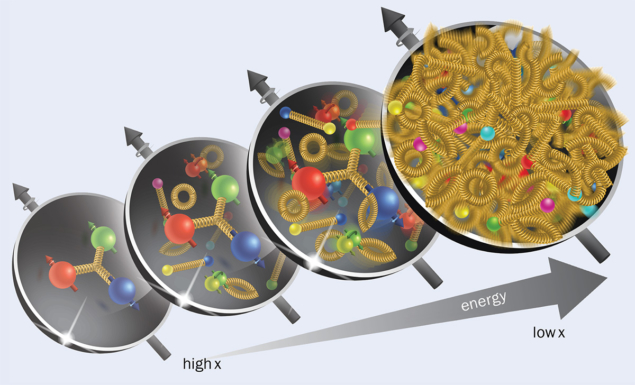
Nuclear matter is inherently complex because the interactions and structures therein are inextricably mixed up: its constituent quarks are bound by gluons that also bind themselves. Consequently, the observed properties of nucleons and nuclei, such as their mass and spin, emerge from a dynamical system governed by quantum chromodynamics (QCD). The quark masses, generated via the Higgs mechanism, only account for a tiny fraction of the mass of a proton, leaving fundamental questions about the role of gluons in the structure of nucleons and nuclei still unanswered.
The underlying nonlinear dynamics of the gluon’s self-interaction is key to understanding QCD and fundamental features of the strong interactions such as dynamical chiral symmetry-breaking and confinement. Yet despite the central role of gluons, and the many successes in our understanding of QCD, the properties and dynamics of gluons remain largely unexplored.
If that’s the back-story, the future is there to be written by the EIC, a unique machine that will enable physicists to shed light on the many open questions in modern nuclear physics.
Back to basics
At the fundamental level, the way in which a nucleon or nucleus reveals itself in an experiment depends on the kinematic regime being probed. A dynamic structure of quarks and gluons is revealed when probing nucleons and nuclei at higher energies, or with higher resolutions. Here, the nucleon transforms from a few-body system, with its structure dominated by three valance quarks, to a regime where it is increasingly dominated by gluons generated through gluon radiation, as discovered at the former HERA electron–proton collider at DESY. Eventually, the gluon density becomes so large that the gluon radiation is balanced by gluon recombination, leading to nonlinear features of the strong interaction.
The LHC and RHIC have shown that neutrons and protons bound inside nuclei already exhibit the collective behaviour that reveals QCD substructure under extreme conditions, as initially seen with high-energy heavy-ion collisions. This has triggered widespread interest in the study of the strong force in the context of condensed-matter physics, and the understanding that the formation and evolution of the extreme phase of QCD matter is dominated by the properties of gluons at high density.
The subnuclear genetic code
The EIC will enable researchers to go far beyond the present one-dimensional picture of nuclei and nucleons, where the composite nucleon appears as a bunch of fast-moving (anti-)quarks and gluons whose transverse momenta or spatial extent are not resolved. Specifically, by correlating the information of the quark and gluon longitudinal momentum component with their transverse momentum and spatial distribution inside the nucleon, the EIC will enable nuclear femtography.
Such femtographic images will provide, for the first time, insight into the QCD dynamics inside hadrons, such as the interplay between sea quarks and gluons. The ultimate goal is to experimentally reconstruct and constrain the so-called Wigner functions – the quantities that encode the complete tomographic information and constitute a QCD “genetic map” of nucleons and nuclei.
• Adapted from “Electron–ion collider on the horizon” by Elke-Caroline Aschenauer, BNL, and Rolf Ent, JLab.
The construction project is led by a joint BNL–JLab management team that integrates the scientific, engineering and management capabilities of JLab into the BNL design effort. JLab, for its part, leads on the design and construction of SRF and cryogenics systems, the energy-recovery linac and several of the electron injector and storage-ring subsystems within the EIC accelerator complex.
More broadly, BNL and JLab are gearing up to work with US and international partners to meet the technical challenges of the EIC in a cost-effective, environmentally responsible manner. The goal: to deliver a leading-edge research facility that will build upon the current CEBAF and RHIC user base to ensure engagement – at scale – from the US and international nuclear-physics communities.
As such, the labs are jointly hosting the EIC experiments in the spirit of a DOE user facility for fundamental research, while the BNL–JLab management team coordinates the engagement of other US and international laboratories into a multi-institutional partnership for EIC construction. Work is also under way with prospective partners to define appropriate governance and operating structures to enhance the engagement of the user community with the EIC experimental programme.
With international collaboration hard-wired into the EIC’s working model, the EIC User Group has been in the vanguard of a global effort to develop the science goals for the facility – as well as the experimental programme to realise those goals. Most importantly, the group has carried out intensive studies over the past two years to document the measurements required to deliver EIC’s physics objectives and the resulting detector requirements. This work also included an exposition of evolving detector concepts and a detailed compendium of candidate technologies for the EIC experimental programme.
Cornerstone collaborations
The resulting Yellow Report, released in March 2021, provides the basis for the ongoing discussion of the most effective implementation of detectors, including the potential for complementary detectors in the two possible collision points as a means of maximising the scientific output of the EIC facility (see “Detectors deconstructed”). Operationally, the report also provides the cornerstone on which EIC detector proposals are currently being developed by three international “proto-collaborations”, with significant components of the detector instrumentation being sourced from non-US partners.
The EIC represents a fundamental link in the chain of continuous R&D and knowledge transfer
Along every coordinate, it’s clear that the EIC project profits enormously from its synergies with accelerator and detector R&D efforts worldwide. To reinforce those benefits, a three-day international workshop was held in October 2020, focusing on EIC partnership opportunities across R&D and construction of accelerator components. This first Accelerator Partnership Workshop, hosted by the Cockcroft Institute in the UK, attracted more than 250 online participants from 26 countries for a broad overview of EIC and related accelerator-technology projects. A follow-up workshop, scheduled for October 2021 and hosted by the TRIUMF Laboratory in Canada, will focus primarily on areas where advanced “scope of work” discussions are already under way between the EIC project and potential partners.
Nurturing talent
While discussion and collaboration between the BNL and JLab communities were prioritised from the start of the EIC planning process, a related goal is to get early-career scientists engaged in the EIC physics programme. To this end, two centres were created independently: the Center for Frontiers in Nuclear Science (CFNS) at Stony Brook University, New York, and the Electron-Ion Collider Center (EIC2) at JLab.
The CFNS, established jointly by BNL and Stony Brook University in 2017, was funded by a generous donation from the Simons Foundation (a not-for-profit organisation that supports basic science) and a grant from the State of New York. As a focal point for EIC scientific discourse, the CFNS mentors early-career researchers seeking long-term opportunities in nuclear science while simultaneously supporting the formation of the EIC’s experimental collaborations.
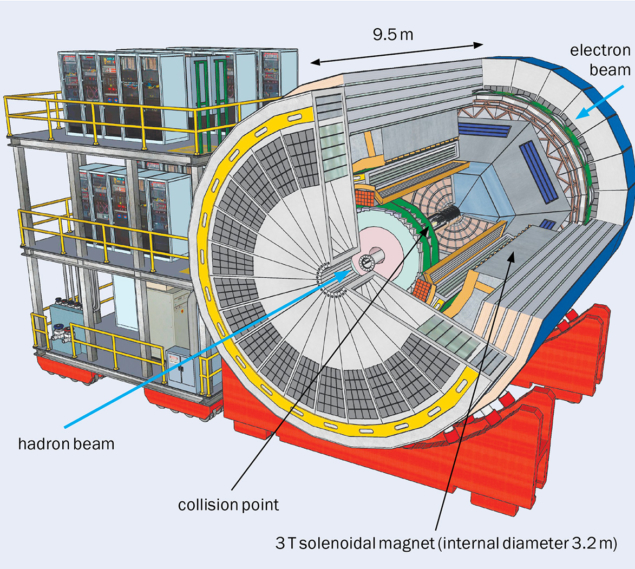
Core CFNS activities include EIC science workshops, short ad-hoc meetings (proposed and organised by members of the EIC User Group), alongside a robust postdoctoral fellow programme to guide young scientists in EIC-related theory and experimental disciplines. An annual summer school series on high-energy QCD also kicked off in 2019, with most of the presentations and resources from the wide-ranging CFNS events programme available online to participants around the world.
In a separate development, the CFNS recently initiated a dedicated programme for under-represented minorities (URMs). The Edward Bouchet Initiative provides a broad portfolio of support to URM students at BNL, including grants to pursue masters or doctoral degrees at Stony Brook on EIC-related research.
Meanwhile, the EIC2 was established at JLab with funding from the State of Virginia to involve outstanding JLab students and postdocs in EIC physics. Recognising that there are many complementary overlaps between JLab’s current physics programme and the physics of the future EIC, the EIC2 provides financial support to three PhD students and three postdocs each year to expand their current research to include the physics that will become possible once the new collider comes online.
Beyond their primary research projects, this year’s cohort of six EIC2 fellows worked together to organise and establish the first EIC User Group Early Career workshop. The event, designed specifically to highlight the research of young scientists, was attended by more than 100 delegates and is expected to become an annual part of the EIC User Group meeting.
The future, it seems, is bright, with CFNS and EIC2 playing their part in ensuring that a diverse cadre of next-generation scientists and research leaders is in place to maximise the impact of EIC science over the decades to come.