The development at CERN of magnesium diboride cables and other advanced superconducting systems for the High-Luminosity LHC is also driving applications beyond fundamental research, describes Amalia Ballarino.
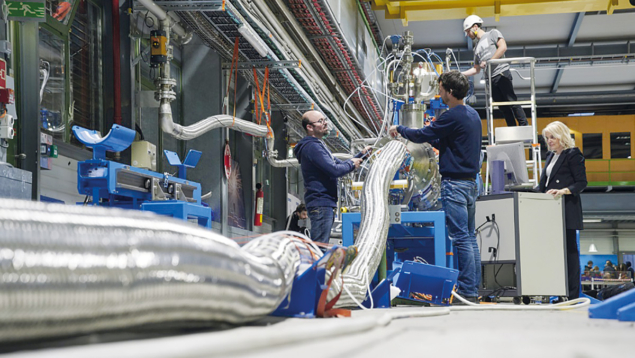
The era of high-temperature superconductivity started in 1986 with the discovery, by IBM researchers Georg Bednorz and Alex Muller, of superconductivity in a lanthanum barium copper oxide. This discovery was revolutionary: not only did the new, brittle superconducting compound belong to the family of ceramic oxides, which are generally insulators, but it had the highest critical temperature ever recorded (up to 35 K, compared with about 18 K in conventional superconductors). In the following years, scientists discovered other cuprate superconductors (bismuth–strontium–copper oxide and yttrium–barium–copper oxide) and achieved superconductivity at temperatures above 77 K, the boiling point of liquid nitrogen (see “Heat is rising” figure). The possibility of operating superconducting systems with inexpensive, abundant and inert liquid nitrogen generated tremendous enthusiasm in the superconducting community.
Several applications of high-temperature superconducting materials with a potentially high impact on society were studied. Among them, superconducting transmission lines were identified as an innovative and effective solution for bulk power transmission. The unique advantages of superconducting transmission are high capacity, very compact volume and low losses. This enables the sustainable transfer of up to tens of GW of power at low and medium voltages in narrow channels, together with energy savings. Demonstrators have been built worldwide in conjunction with industry and utility companies, some of which have successfully operated in national electricity grids. However, widespread adoption of the technology has been hindered by the cost of cuprate superconductors.
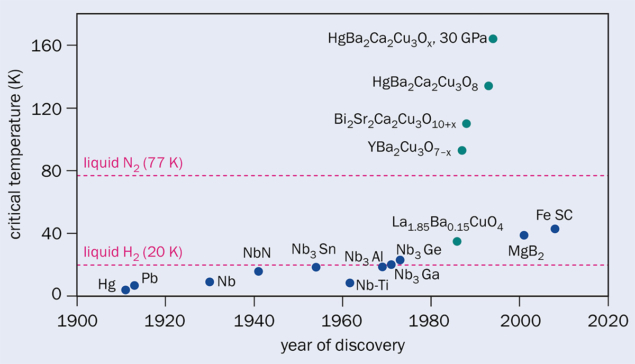
In particle physics, superconducting magnets allow high-energy beams to circulate in colliders and provide stronger fields for detectors to be able to handle higher collision energies. The LHC is the largest superconducting machine ever built, and the first to also employ high-temperature superconductors at scale. Realising its high-luminosity upgrade and possible future colliders is driving the use of next-generation superconducting materials, with applications stretching far beyond fundamental research.
High-temperature superconductivity (HTS) was discovered at the time when the conceptual study for the LHC was ongoing. While the new materials were still in a development phase, the potential of HTS for use in electrical transmission was immediately recognised. The powering of the LHC magnets (which are based on the conventional superconductor niobium titanium, cooled by superfluid helium) requires the transfer of about 3.4 MA of current, generated at room temperature, in and out of the cryogenic environment. This is done via devices called current leads, of which more than 3000 units are installed at different underground locations around the LHC’s circumference. The conventional current–lead design, based on vapour-cooled metallic conductors, imposes a lower limit (about 1.1 W/kA) on the heat in-leak into the liquid helium. The adoption of the HTS BSCCO 2223 (bismuth–strontium–calcium copper oxide ceramic) tape – operated in the LHC current leads in the temperature range 4.5 to 50 K – enabled thermal conduction and ohmic dissipation to be disentangled. Successful multi-disciplinary R&D followed by prototyping at CERN and then industrialisation, with series production of the approximately 1100 LHC HTS current leads starting in 2004, resulted in both capital and operational savings (avoiding one extra cryoplant and an economy of about 5000 l/h of liquid helium). It also encouraged wider adoption of BSCCO 2223 current–lead technology, for instance in the magnet circuits for the ITER tokamak, which benefit via a collaboration agreement with CERN on the development and design of HTS current leads.
MgB2 links at the HL-LHC
The discovery of superconductivity in magnesium diboride (MgB2) in 2001 generated new enthusiasm for HTS applications. This material, classified as medium-temperature superconductor, has remarkable features: it has a critical temperature (39 K) some 30 K higher than that of niobium titanium, a high current density (to date in low and medium magnetic fields) and, crucially, it can be industrially produced as round multi-filamentary wire in long (km) lengths. These characteristics, along with a cost that is intrinsically lower than other available HTS materials, make it a promising candidate for electrical applications.
At the LHC the current leads are located in the eight straight sections. For the high-luminosity upgrade of the LHC (HL-LHC), scheduled to be operational in 2029, the decision was taken to locate the power converters in new, radiation-free underground technical galleries above the LHC tunnel. The distance between the power converters and the HL-LHC magnets spans about 100 m and includes a vertical path via an 8 m shaft connecting the technical galleries and the LHC tunnel. The large current to be transferred across such distance, the need for compactness, and the search for energy efficiency and potential savings led to the selection of HTS transmission as the enabling technology.
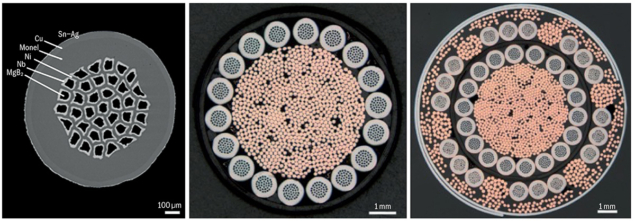
The electrical connection, at cryogenic temperature, between the HL-LHC current leads and the magnets is performed via superconducting links based on MgB2 technology. MgB2 wire is assembled in cables with different layouts to transfer currents ranging from 0.6 kA to 18 kA. The individual cables are then arranged in a compact assembly that constitutes the final cable feeding the magnet circuits of either the HL-LHC inner triplets (a series of quadrupole magnets that provides the final focusing of the proton beams before collision in ATLAS and CMS) or the HL-LHC matching sections (which match the optics in the arcs to those at the entrance of the final-focus quadrupoles), and the final cable is incorporated in a flexible cryostat with an external diameter of up to 220 mm. The eight HL-LHC superconducting links are about 100 m long and transfer currents of about 120 kA for the triplets and 50 kA for the matching sections at temperatures up to 25 K, with cryogenic cooling performed with helium gas.
The R&D programme for the HL-LHC superconducting links started in around 2010 with the evaluation of the MgB2 conductor and the development, with industry, of a round wire with mechanical properties enabling cabling after reaction. Brittle superconductors, such as Nb3Sn – used in the HL-LHC quadrupoles and also under study for future high-field magnets – need to be reacted into the superconducting phase via heat treatments, at high temperatures, performed after their assembly in the final configuration. In other words, those conductors are not superconducting until cabling and winding have been performed. When the R&D programme was initiated, industrial MgB2 conductor existed in the form of multi-filamentary tape, which was successfully used by ASG Superconductors in industrial open MRI systems for transporting currents of a few hundred amperes. The requirement for the HL-LHC to transfer current to multiple circuits for a total of up to 120 kA in a compact configuration, with multiple twisting and transposition steps necessary to provide uniform current distribution in both the wires and cables, called for the development of an optimised multi-filamentary round wire.
Carried out in conjunction with ASG Superconductors, this development led to the introduction of thin niobium barriers around the MgB2 superconducting filaments to separate MgB2 from the surrounding nickel and avoid the formation of brittle MgB2–Ni reaction layers that compromise electro-mechanical performance; the adoption of higher purity boron powder to increase current capability; the optimisation in the fraction of Monel (a nickel-copper alloy used as the main constituent of the wire) in the 1 mm-diameter wire to improve mechanical properties; the minimisation of filament size (about 55 µm) and twist pitch (about 100 mm) for the benefit of electro-mechanical properties; the addition of a copper stabiliser around the Monel matrix; and the coating of tin–silver onto the copper to ensure the surface quality of the wire and a controlled electrical resistance among wires (inter-strand resistance) when assembled into cables. After successive implementation and in-depth experimental validation of all improvements, a robust 1 mm-diameter MgB2 wire with required electro-mechanical characteristics was produced.

The next step was to manufacture long unit lengths of MgB2 wire via larger billets (the assembled composite rods that are then extruded and drawn down in a long wire). The target unit length of several kilometres was reached in 2018 when series procurement of the wire was launched. In parallel, different cable layouts were developed and validated at CERN. This included round MgB2 cables in a co-axial configuration rated for 3 kA and for 18 kA at 25 K (see “Complex cabling” figure). While the prototypes made at CERN were 20 to 30 m long, the cable layout incorporated, from the outset, characteristics to enable production via industrial cabling machines of the type used for conventional cables. Splice techniques as well as detection and protection aspects were addressed in parallel with wire and cable development. Both technologies are strongly dependent on the characteristics of the superconductor, and are of key importance for the reliability of the final system.
The first qualification at 24 K of a 20 kA MgB2 cable produced at CERN, comprising two 20 m lengths connected together, took place in 2014. This followed the qualification at CERN of short-model cables and other technological aspects, as well as the construction of a dedicated test station enabling the measurement of long cables operated at higher temperatures, in a forced flow of helium gas. The cables were then industrially produced at TRATOS Cavi via a contract with ICAS, in a close and fruitful collaboration that enabled – while operating heavy industrial equipment – the requirements identified during the R&D phase. The complexity of the final cables required a multi-step process that used different cabling, braiding and electrically insulating lines, and the implementation of a corresponding quality-assurance programme. The first industrial cables, which were 60 m long, were successfully qualified at CERN in 2018. Final prototype cables of the type needed for the HL-LHC (for both the triplets and matching sections) were validated at CERN in 2020, when series production of the final cables was launched. As of today, the full series of about 1450 km of MgB2 wire – the first large-scale production of this material – and five of the eight final MgB2 cables needed for the HL-LHC have been produced.
The use of hydrogen can diversify energy sources as it significantly reduces greenhouse-gas emissions and environmental pollution during energy conversion
Superconducting wire and cables are the core of a superconducting system, but the system itself requires a global optimisation, which is achieved via an integrated design. Following this approach, the challenge was to investigate and develop, in industry, long and flexible cryostats for the superconducting links with enhanced cryogenic performance. The goal was to achieve a low static heat load (< 1.5 W/m) into the cryogenic volume of the superconducting cables while adopting a design – a two-wall cryostat without intermediate thermal screen – that simplifies the cooling of the system, improves the mechanical flexibility of the links and eases handling during transport and installation. This development, which ran in parallel with the wire and cable activities, led to the desired results and, after an extensive test campaign at CERN, the developed technology was adopted. Series production of these cryostats is taking place at Cryoworld in the Netherlands.
The optimised system minimises the cryogenic cost for the cooling such that a superconducting link transfers – from the tunnel to the technical galleries – just enough helium gas to cool the resistive section of the current leads and brings it to the temperature (about 20 K) for which the leads are optimised. In other words, the superconducting link does not add cryogenic cost to the refrigeration of the system. The links, which are rated for currents up to 120 kA, are sufficiently flexible to be transported, as for conventional power cables, on drums about 4 m in diameter and can be manually pulled, without major tooling, during installation (see “kA currents” image). The challenge of dealing with the thermal contraction of the superconducting links, which shrink by about 0.5 m when cooled down to cryogenic temperature, was also addressed. An innovative solution, which takes advantage of bends and is compatible with the fixed position of the current lead cryostat, was validated with prototype tests.
Novel HTS leads
Whereas MgB2 cables transfer high DC currents from the 4.5 K liquid helium environment in the LHC tunnel to about 20 K in the HL-LHC new underground galleries, a different superconducting material is required to transfer the current from 20 to 50 K, where the resistive part of the current leads makes the bridge to room temperature. To cope with the system requirements, novel HTS current leads based on REBCO (rare-earth barium copper oxide) HTS superconducting tape – a material still in a development phase at the time of the LHC study – have been conceived, constructed and qualified to perform this task (see “Bridging the gap” image). Compact, round REBCO cables ensure, across a short (few-metre-long) length, the electrical transfer from the MgB2 to 50 K, after which the resistive part of the current leads finally brings the current to room temperature. In view of the complexity of dealing with the REBCO conductor, the corresponding R&D was done at CERN, where a complex dedicated cabling machine was also constructed.
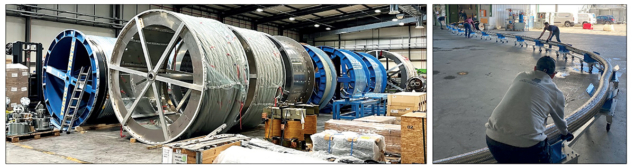
While REBCO tape is procured from industry, the challenges encountered during the development of the cables were many. Specific issues associated with the tape conductor, for example electrical resistance internal to the tape and the dependence of electrical properties on temperature and cycles applied during soldering, were identified and solved with the tape manufacturers. A conservative approach imposing zero critical current degradation of the tape after cabling was implemented. The lessons learnt from this development are also instrumental for future projects employing REBCO conductors, including the development of high-field REBCO coils for future accelerator magnets.
The series components of the HL-LHC cold-powering systems (superconducting links with corresponding terminations) are now in production, with the aim to have all systems available and qualified in 2025 for installation in the LHC underground areas during the following years. Series production and industrialisation were preceded by the completion of R&D and technological validations at CERN. Important milestones have been the test of a sub-scale 18 kA superconducting link connected to a pair of novel REBCO current leads in 2019, and the test of full-cross section, 60 m-long superconducting lines of the type needed for the LHC triplets and for the matching sections, both in 2020.
The complex terminations of the superconducting links involve two types of cryostat that contain, at the 20 K side, the HTS current leads and the splices between REBCO and MgB2 cables and, at the 4.2 K side, the splices between the niobium titanium and the MgB2 cables. A specific development in the design was to increase compactness and enable the connection of the cryostat with the current leads to the superconducting link at the surface, prior to installation in the HL-LHC underground areas (see “End of the line” figure). The series production of the two cryostat terminations is taking place via collaboration agreements with the University of Southampton and Uppsala University.
The displacement of the current leads via the adoption of superconducting links brings a number of advantages. These include freeing precious space in the main collider ring, which becomes available for other accelerator equipment, and the ability to locate powering equipment and associated electronics in radiation-free areas. The latter relaxes radiation-hardness requirements for the hardware and eases access for personnel to carry out the various interventions required during accelerator operations.
Cooling with low-density helium gas also makes electrical transfer across long vertical distances feasible. The ability to transfer high currents from underground tunnels to surface buildings – as initially studied for the HL-LHC – is therefore of interest for future machines, such as the proposed Future Circular Collider at CERN. Flexible superconducting links can also be applied to “push–pull” arrangements of detectors at linear colliders such as the proposed CLIC and ILC, where the adoption of flexible powering lines can simplify and reduce the time for the exchange of experiments sharing the same interaction region.
An enabling technology
Going beyond fundamental research in physics, superconductivity is an enabling technology for the transfer of GWs of power across long distances. The main benefits, in addition to incomparably higher power transmission, are small size, low total electrical losses, minimised environmental impact and more sustainable transmission. HTS offers the possibility of replacing resistive high-voltage overhead lines, operated across thousands of kilometres at voltages reaching about 1000 kV, with lower voltage lines, laid underground with reduced footprints.

Long-distance power transmission using hydrogen- cooled MgB2 superconducting links, potentially associated with renewable energy sources, is identified as one of the leading ways towards a future sustainable energy system. Since hydrogen is liquid at 20 K (the temperature at which MgB2 is superconducting), large amounts can be stored and used as a coolant for superconducting lines, acting at the same time as the energy vector and cryogen. In this direction, CERN participated – at a very early stage of the HL-LHC superconducting links development – in a project launched by Carlo Rubbia as scientific director of the Institute for Advanced Sustainability Studies (IASS) in Potsdam. Around 10 years ago, CERN and IASS joint research culminated in the record demonstration of the first 20 kA MgB2 transmission line operated at liquid hydrogen temperature. This activity continued with a European initiative called BestPaths, which demonstrated a monopole MgB2 cable system operated in helium gas at 20 K. This was qualified in industry for 320 kV operation and at 10 kA at CERN, proving 3.2 GW power transmission capability. This initiative involved European industry and France’s transmission system operator. In Italy, the INFN has recently launched a project called IRIS based on similar technology (see CERN Courier January/February 2023 p9).
In addition to transferring power across long distances with low losses and minimal environmental impact, the development of high-performance, low-cost, sustainable and environmentally friendly energy storage and production systems is a key challenge for society. The use of hydrogen can diversify energy sources as it significantly reduces greenhouse-gas emissions and environmental pollution during energy conversion. In aviation, alternative-propulsion systems are studied to reduce CO2 emission and move toward zero-emission flights. Scaling up electric propulsion to larger aircraft is a major challenge. Superconducting technologies are a promising solution as they can increase power density in the propulsion chain while significantly lowering the mass of the electrical distribution system. In this context, a collaboration agreement has recently been launched between CERN and Airbus UpNext. The construction of a demonstrator of superconducting distribution in aircraft called SCALE (Super-Conductor for Aviation with Low Emissions), which uses the HL-LHC superconducting link technology, was recently launched at CERN.
CERN’s developed experience in superconducting-link technology is also of interest to large data centres, with a collaboration agreement between CERN and Meta under discussion. The possibility of locating energy equipment remotely from servers, of transferring efficiently large power in a compact volume, and of meeting sustainability goals by reducing carbon footprints are motivating a global re-evaluation of conventional systems in light of the potential of superconducting transmission.
Such applications demonstrate the virtuous circle between fundamental and applied research. The requirements of fundamental exploration in particle physics research have led to the development of increasingly powerful and sophisticated accelerators. In this endeavour, scientists and engineers engage in developments initially conceived to address specific challenges. This often requires a multi-disciplinary approach and collaboration with industry to transform prototypes into mature technology ready for large-scale application. Accelerator technology is a key driver of innovation that may also have a wider impact on society. The superconducting-link system for the HL-LHC project is a shining example.