Strong electric fields in crystals provide a laboratory to study QED.

Image credit: Mikkel D Lund/NA63.
“Why still do experimental quantum electrodynamics, isn’t everything known?” This provocative question is often heard by the collaborators at one of the smaller CERN experiments, NA63. Their answer is almost as short as the question: it is precisely the fact that everything is supposed to be known that makes it interesting. This understanding enables the exploration of physics in regimes of strong electromagnetic fields, for example as a function of interaction times or in studies of scattering. The results cast light on phenomena in various branches of physics.
Take, as an example, the emission of beamstrahlung, which is expected in the next generation of electron–positron linear colliders, such as the Compact Linear Collider (CLIC) currently under conceptual design at CERN. Particles in a bunch of particles in one beam “see” the electric field in the opposing bunch as boosted by 2γ2–1, where γ is the Lorentz factor. This appears as a strong electric field in the bunch’s rest frame and leads to the emission of intense synchrotron-like radiation, which is known as beamstrahlung. The electric field seen by the particles is comparable to the so-called critical field, which depends only on the reduced Planck’s constant, ħ–, the speed of light, c, and the mass, m, and charge, e, of the electron – m2c3/h–e – and is equivalent to 1.32 × 1016 V/cm and a corresponding magnetic field of 4.41 × 109 T. In such fields, quantum corrections to the emission of synchrotron radiation become important in determining the emission spectrum. They lead to a strong suppression when compared with the classical calculations that are applicable in most other contexts for synchrotron radiation emission.
Into the laboratory
The effects of strong fields are also relevant in many other branches, ranging from the so-called “bubble-regime” in plasma wakefields used for extremely high-gradient particle acceleration, through astrophysical objects such as magnetars, to intense lasers and heavy-ion collisions. The concept even applies in a gravitational analogue – Hawking radiation. Therefore, further investigation of the underlying phenomena is of broad interest.
Clearly, electric fields of the order the critical field are inaccessible in the laboratory. However, by replacing the opposing bunch in the example of a linear collider by a crystalline target, processes linked to the critical field can be studied with relative ease because the crystalline electric fields are orders of magnitude higher. At small angles of incidence to a crystallographic axis or plane, the strong electric fields of the nuclear constituents add coherently to form a macroscopic, continuous field with a peak value around 1011 V/cm. In the rest frame of an ultra-relativistic electron with γ around 105, the field encountered by the incident particle thus becomes comparable to the critical field.
Applications of these strong crystalline electric fields are widely known, in particular in “channelling”, where a beam of charged particles is steered by the fields within a crystal. This has been used, for example in the NA48 experiment at CERN, to deflect a well defined fraction of the main proton beam for the generation of kaons.
The NA63 experiment, following on from its predecessor, NA43, focuses on fundamental investigations of the strong fields themselves. The results have already shown that the emission of synchrotron radiation in the quantum regime is, indeed, well understood, being strongly suppressed as expected. These results mean that reliable estimates based on QED of beamstrahlung in future machines can now be made. In addition, the spin-flip component of the synchrotron-like radiation that is emitted as the beam passes through the crystal is many orders of magnitude higher in energy and intensity than that of a storage ring, with corresponding polarization times of femtoseconds instead of hours.
Strong scattering effects
The suppression in the emission of radiation arises loosely speaking because the field becomes so strong that the particle is deviated out of the formation zone necessary for the generation of the photon – in effect before it has time to generate the radiation. It is equivalent to a shortening of the formation zone. Although the concept of the formation zone was introduced more than 50 years ago by the Armenian physicist Mikhail Ter-Mikaelian, it is still a surprise to many that it can take time corresponding to macroscopic travel distances for a relativistic electron to emit a photon. This is the basis of the Landau-Pomeranchuk-Migdal (LPM) effect, where multiple scattering within the formation length leads to a reduction in radiation emission.

Image credit: K K Andersen/NA63.
Figure 1 illustrates the suppression mechanism at play. It depicts the electric field from a particle, incident along the dashed line, that has scattered twice (at locations marked by crosses). Outside a radius given by the time since the scattering event, the field points towards the location that the particle would have had if it had not scattered. This is a result of the finite propagation time of information; inside the corresponding sphere, the field follows the particle. The transverse components correspond to radiation and, because of the short time between the scattering events, they are closely spaced and pointing in opposite directions. A distant observer looking at low frequencies will see two electric field lines that mutually cancel – and, therefore, less radiation. It is as if a “semi-bare” electron is interacting.
However, as the NA63 collaboration has recently shown, if a particle impinges on a target that is so thin that the formation zone extends beyond the target, then the LPM suppression is alleviated. To study this effect the collaboration measured the radiation emission from ultra-relativistic electrons in targets consisting of a number of thin foils of tantalum corresponding to 0.03%–5% radiation lengths. They found that, for the thinnest targets, the radiation emission agrees with expectations from the Bethe-Heitler formulation of bremsstrahlung, with the target acting as a single scatterer. Only as the thickness increases does the distorted Coulomb field resulting from the first scattering lead to a suppression of radiation emission in subsequent scattering such that the radiation yield becomes a logarithmic function of the thickness, eventually to become LPM suppression (Thomsen et al. 2010).
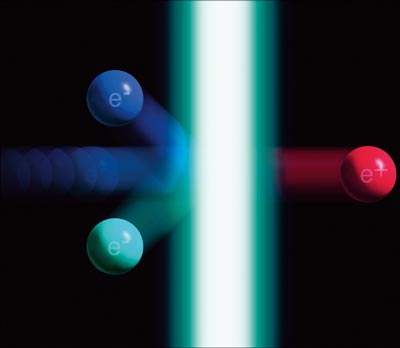
Image credit: Bent A Lomholt.
The NA63 collaboration has also studied higher-order processes, such as “trident production”, in which an electron impinging on an electromagnetic field produces a positron–electron pair directly through the emission of a virtual photon. The process is illustrated in figure 2 in a reference frame close to the rest frame of the incident electron, in which the field has the critical value. In the laboratory frame, the original particle plus the pair are all directed forwards in a three-prong pattern, giving rise to the name “trident”. The effect is reminiscent of a phenomenon studied by Oskar Klein and Fritz Sauter 80 years ago – the so-called Klein paradox. Klein was one of the first to do calculations using the celebrated equation of Paul Dirac. In 1929 Klein looked at the probability of reflection of an electron from the steep potential barrier provided by an electric field and found that the probability for transmission into a potential of infinite height approached the velocity of the incident electron in units of the speed of light, i.e. that transmission into a “forbidden” region approaches certainty. Soon after, Sauter found that the process takes place for electric fields beyond the critical field, i.e. when the field is so high that an electron transported over a Compton wavelength produces its rest mass, mc2. Today, this process is understood in terms of pair production at the boundary, but without knowledge of the positron this was an impossible conclusion for Klein, hence the name “Klein paradox”.

Image credit: NA63 collaboration.
Studies by NA63 of trident production, with crystals of germanium a few hundred micrometres thick, have shown a similar phenomenon: that when the crystal is turned to an axial direction along the beam, giving rise to a critical field in the particle’s rest frame, the trident process increases significantly (Esberg et al. 2010). Recent calculations have shown that trident production is an important factor in the design of the collision zone at CLIC, underlining the relevance of these experimental investigations.
A suppression mechanism also occurs in the case of pair production. In this case mutual screening of the charges in the pair substantially reduces the energy deposition in matter in the vicinity of the creation vertex. Because of the directionality of the pair, at high energies this internal screening – the King-Perkins-Chudakov effect – takes place over a distance of several tens of micrometres. This is a distance comparable to the sensitive layers in a CCD or a silicon vertex detector (figure 3), which can be used to study the effect.
Finally, as Allan Sørensen of Aarhus University has recently calculated, bremsstrahlung from relativistic heavy ions is expected to show a peak-structure connected to the finite size of the nucleus. The detection of this effect is among the future plans of NA63.
So QED still presents challenges, even for the otherwise well known case of radiation emission. In the words of one of the originators of the quantum theory of beamstrahlung, Richard Blankenbecler: “It is surprising that there is so much more to learn about such a well understood process.”