Assembly of the tokamak that will confine a 150-million-degree plasma inside the ITER fusion experiment marks a crucial new phase in the project, describes Tim Luce.

At the heart of the ITER fusion experiment is an 18 m-tall, 1000-tonne superconducting solenoid – the largest ever built. Its 13 T field will induce a 15 MA plasma current inside the ITER tokamak, initiating a heating process that ultimately will enable self-sustaining fusion reactions. Like all-things ITER, the scale and power of the central solenoid is unprecedented. Fabrication of its six niobium-tin modules began nearly 10 years ago at a purpose-built General Atomics facility in California. The first module left the factory on 21 June and, after traveling more than 2400 km by road and then crossing the Atlantic, the 110 tonne component arrived at the ITER construction site in southern France on 9 September. During a small ceremony marking the occasion, the director of engineering and projects for General Atomics described the job as: “among the largest, most complex and demanding magnet programmes ever undertaken” and “the most important and significant project of our careers.”
The US is one of seven ITER members, along with China, the European Union, India, Japan, Korea and Russia, who ratified an international agreement in 2007. Each member shares in the cost of project construction, operation and decommissioning, and also in the experimental results and any intellectual property. Europe is responsible for the largest portion of construction costs (45.6%), with the remainder shared equally by the other members. Mirroring the successful model of collider experiments at CERN, the majority (85%) of ITER-member contributions are to be delivered in the form of completed components, systems or buildings – representing untold hours of highly skilled work both in the member states and at the ITER site.
First plasma
Assembly of the tokamak, which got under way in 2020, marks an advance to a crucial new phase for the ITER project. Production of its 18 D-shaped coils that provide the toroidal magnetic field, each 17 m high and weighing 350 tonnes, is in full swing, while its circular poloidal coils are close to completion. The remaining solenoid modules and all other major tokamak components are scheduled to be on site by mid-2023. Despite the impact of the global pandemic, the ITER teams are working towards the baseline target for “first plasma” by the end of 2025, with more than 2000 persons on site each day.
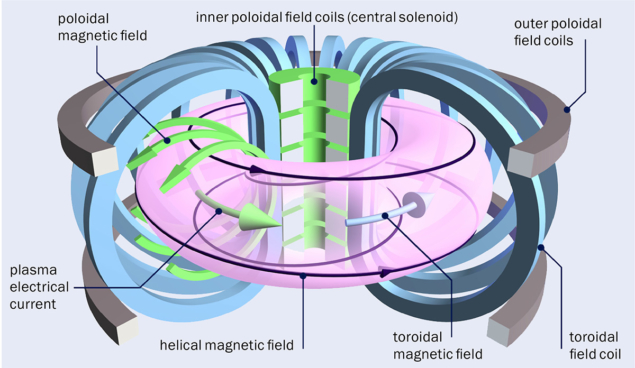
ITER’s purpose is to demonstrate the scientific and technological feasibility of fusion power for peaceful purposes. Key objectives are defined for this demonstration, namely: production of 500 MW of fusion power with a ratio of fusion power to input heating power (Q) of at least 10 for at least 300 seconds, and sustainment of fusion power with Q = 5 consistent with steady-state operation. The key factor in reaching these objectives is the world’s largest tokamak, a concept whose name comes from a Russian acronym roughly translated “toroidal chamber with magnetic coils”. This could also describe CERN’s Large Hadron Collider (LHC), but as we will see, the two magnetic confinement schemes are significantly different.
Among the largest, most complex and demanding magnet programmes ever undertaken
ITER chose deuterium and tritium (heavier variants of ordinary hydrogen) for its fuel because the D–T cross-section is the highest of all known fusion reactions. However, the energy at which the cross-section is maximum (~65 keV) is equivalent to almost 1 billion degrees. As a result, the fuel will no longer be in the form of gas as it is introduced but in the plasma state, where it is broken down to its electrically charged components (ions and electrons). As in the LHC, the electric charge introduces the possibility to hold the ions and electrons in place using magnetic fields generated by electromagnets – in both cases by superconducting magnets held at temperatures near absolute zero to avoid massive electrical consumption.

A simple picture of how the magnets in ITER work together to confine a plasma with temperatures greater than 100 million degrees begins with the toroidal field coils (see “Trapping a plasma” figure). Eighteen of these are arranged to make a magnetic field that is circular-centered on a vertical line. Charged particles, to the crudest approximation, follow the magnetic field, so it would seem that the problem of confining them is solved. However, at the next level of approximation, the charged particles actually make small “gyro-orbits”, like beads on a wire. This introduces a difficulty because the “gyroradius” of these orbits depends on the strength of the magnetic field, and the toroidal magnetic field increases in strength closer to the vertical line defining its centre. This means that the gyroradius is smaller on the inner part of the orbit, which leads to a vertical motion of the charged particles. Since the direction of motion depends on the charge of the particle, however, the opposite charges move away from each other. This makes a vertical electric field which, when combined with the toroidal field, rapidly expels charged particles radially outward – eliminating confinement! Two Russian physicists, Tamm and Sakharov, proposed the idea in the 1950s that a current flowing in the plasma in the toroidal direction would generate a net helical field and charged particles flowing along the total field would short out the electric field, leading to confinement. This was the invention of the tokamak magnetic confinement concept.
Magnetic configuration
In ITER, this current is generated by the powerful central solenoid, aligned on the vertical line at the centre of the toroidal field. It acts as the primary winding of a transformer, with the plasma as the secondary. There remains one more issue to address, again with magnets. The pressure and current in the plasma result in a force that tries to push the plasma further from the vertical line at the centre. To counter this force in ITER, six “poloidal field” coils are aligned – again about the vertical centerline – to generate vertical fields that push the plasma back toward the vertical line and also shape the plasma in ways that enhance the performance. A number of correction coils will complete ITER’s complex magnetic configuration, which will demonstrate the deployment of the Nb3Sn conductor – the same as is being implemented for high-field accelerator magnets at the High-Luminosity LHC and as proposed for future colliders – on a massive scale. CERN signed a collaboration agreement with ITER in 2008 concerning the design of high-temperature superconducting current leads and other magnet technologies, and acted as one of the “reference” laboratories for testing ITER’s superconducting strands.
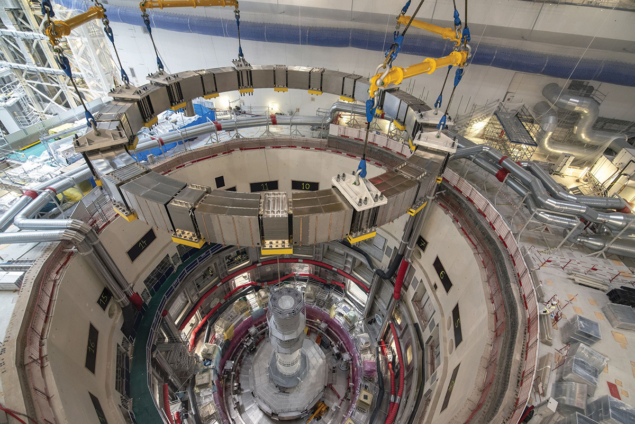
Despite the pandemic disrupting production and transport, the first step of ITER’s tokamak assembly sequence – the installation of the base of the cryostat into the tokamak bioshield – was achieved in May 2020. The ITER cryostat, which must be made of non-magnetic stainless steel, will keep the entire (30 m diameter by 30 m high) tokamak assembly at the low temperatures necessary for the magnets to function. It comes in four pieces (base, lower and upper cylinders, and lid) that are welded together in the tokamak building. At 1250 tonnes, the cryostat-base lift was the heaviest of the entire assembly sequence, its successful completion officially starting the assembly sequence (see “Heavy lifting” image). Later in 2020, the lower cylinder was then installed and welded to the base.
Bottle up
With the “bottle” to hold the tokamak placed in position, installation of the electromagnets could begin. The two poloidal field coils at the bottom of the tokamak, PF6 and PF5, had to be installed first. PF6 was placed inside the cryostat earlier this year (see “Poloidal descent” image), while the second was lifted into place this September. The next big milestone is the assembly and installation of the first “sector” of the tokamak. The vacuum vessel in which the fusion plasma is made is divided into nine equal sectors (like the slices of an orange), due to limitations on the lifting capacity and to facilitate parallel fabrication of these large objects. Each sector of the vacuum vessel (see “Monster moves” image) has two toroidal field coils associated with it.

In August, this vacuum vessel and its associated thermal shields were assembled together with the toroidal field coils on the sector sub-assembly tool for the first time (see “Shaping up” image). Once joined into a single unit, it will be installed in the cryostat in late 2021. The second vacuum-vessel sector arrived on site in August and will be assembled with the two associated toroidal-field coils already on site, with a target to install the final unit in the cryostat early in 2022. Sector components are scheduled to arrive, be put together, and then installed in the cryostat and welded together in assembly-line fashion, with the closure of the vacuum vessel scheduled for the end of 2023. The six central-solenoid modules are also to be assembled outside the cryostat into a single structure and installed in the cryostat shortly before closure. Following the arrival of the first module this summer, the second is complete and ready for shipping. Of the remaining four niobium-titanium poloidal field magnets, three are being fabricated on-site because they are too large to transport by road and all four are in advanced stages of production.
Of course, there is more to ITER than its tokamak. In parallel, work on the supporting plant is under way. Four large transformers, which draw the steady-state electrical power from the grid, have been in operation since early 2019, while the medium- and low-voltage load centres that power clients in the plant buildings have been turned over to the operations division. The secondary and tertiary cooling systems, the chilled water and demineralised water plants, and the compressed-air and breathable-air plants are also currently being commissioned. The three large transformers that connect the pulsed power supplies for the magnets and the plasma heating systems have been qualified for operation on the 400 kV grid. The next big steps are the start of functional testing of the cryoplant and the reactive power compensation at the end of this year, and of the magnet power supplies and the first plasma heating system early in 2022.

Perhaps the most common question one encounters when talking about ITER is: when will tokamak operations begin? Following the closure of the vacuum vessel in 2023, the current baseline schedule includes one year of installation work inside the cryostat before its closure, followed by integrated commissioning of the tokamak in 2025, culminating in “first plasma” by the end of 2025. By mandate from ITER’s governing body, the ITER Council, this schedule was put into place in 2016 as the “fastest technically achievable”, meaning no contingency. Clearly the pandemic has impacted the ability to meet that schedule, but the actual impact is still not possible to determine accurately. The challenge in this assessment is that 85% of the ITER components are delivered as in-kind contributions from the ITER members, and the pandemic has affected and continues to affect the manufacturing work on items that take years to complete. The components now being installed were substantially complete at the onset of the pandemic, but even these deliveries have encountered difficulties due to the disruption of the global shipping industry. Component installation in the tokamak complex has also been impacted by limited availability of components, goods and services. The possibility of recovery actions or further restrictions is not possible to predict with the needed accuracy today. In this light, the ITER Council has challenged us to do the best possible effort to maintain the baseline schedule, while preparing an assessment of the impact for consideration of a revised baseline schedule next year. The ITER Organization, domestic agencies in the ITER members responsible for supplying in-kind components, and contractors and suppliers around the world are working together to meet this additional challenge.
What the future holds
ITER is expected to operate for 20 years, providing crucial information about both the science and the technology necessary for a fusion power plant. For the science, beyond the obvious interest in meeting ITER’s performance objectives, qualitative frontiers will be crossed in two essential areas of plasma physics. First, ITER will be the first “burning” plasma, where the dominant heating power to sustain the fusion output comes directly from fusion itself. Aspects of the relevant physics have been studied for many years, but the operating point of ITER places it in a fundamentally different regime from present experiments. The same is true of the second frontier: the handling of heat and particle exhaust in ITER. There is a qualitative difference predicted by our best simulation capabilities between the ITER operating point and present experiments. This is also the first touch-point between the physics and the technology: the physics must enable the survival of the wall, while the wall must allow the plasma physics to yield the conditions needed for the fusion reactions. Other essential technologies such as the means to make new fusion fuel (tritium), recycling of the fuel in use in real-time and remote handling for maintenance activities will all be pioneered in ITER.
ITER will provide crucial information about both the science and technology necessary for a fusion power plant
While ITER will demonstrate the potential for fusion energy to become the dominant source of energy production, harnessing that potential requires the demonstration not just of the scientific and technical capabilities but of the economic feasibility too. The next steps along that path are true demonstration power plants – “DEMOs” in fusion jargon – that explore these steps. ITER members are already exploring DEMO options, but no commitments have yet been made. The continuing advance of ITER is critical not just to motivate these next steps but also as a vision of a future where the world is powered by an energy source with universally available fuel and no impact on the environment. What a tremendous gift that would be for future generations.