The first joint publications by H1 and ZEUS start a new harvest of results.
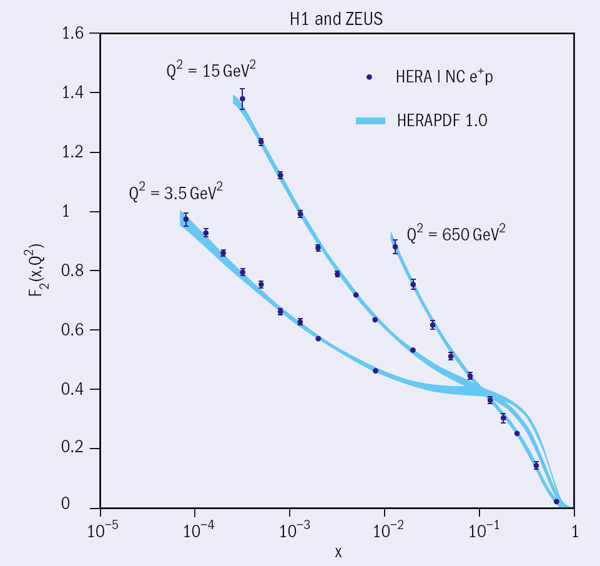
High-energy physics experiments address fundamental questions using large facilities and complex detectors, which often use innovative detection techniques. It is usual to build and operate more than one such detector at the same accelerator – to confront, compare and eventually merge the measurements. Combining measurements made by similar detectors becomes feasible and ultimately mandatory when these detectors are well understood and tested with many physics analyses. This step was achieved recently by the H1 and ZEUS experiments, which took data at DESY’s HERA collider from 1992 until 2007.
HERA was the only electron–proton collider ever built, providing collisions between electrons or positrons of 27.5 GeV and protons of up to 920 GeV to give a centre-of-mass energy of 320 GeV. The data collected at HERA are unique and have led to precise measurements of the proton structure, in particular in the region of low Bjorken-x, below 0.01, where no other measurement exists. At HERA, the point-like electron probes the gluon-fabric of the proton down to scales as small as 1/1000 of the proton’s radius. These measurements provide a clean testing ground for the Standard Model. Furthermore, searches for new physics signals at HERA are complementary to searches made at other colliders.
So far, H1 and ZEUS have published individual measurements investigating a plethora of different processes in more than 400 scientific articles. However, for the first time, three joint publications have recently been submitted to the Journal of High Energy Physics. These combinations of the H1 and ZEUS data in coherent analyses address a new paradigm in this field of research.
Universal structure functions
Combining data sets improves individual measurements because the amount of information increases. The theory of statistics states that uncertainties diminish by a factor of √2 when the amount of data is doubled. When systematic uncertainties are taken into account, however, the effects are more subtle: there is no gain for errors correlated between the experiments. Typical examples of this kind are theoretical calculations that are needed to extract the experimental results. Uncertainties that are fully uncorrelated (not only between the experiments but also from one measurement point to the next) have a similar behaviour to statistical errors and are reduced by the magical factor of √2. Finally, the most interesting case comes from errors that are correlated within each experiment, but uncorrelated between experiments. One example is the energy scale of the calorimetric measurements: the technologies of the calorimeters in the two experiments are different and they are calibrated using independent procedures. Hence the respective errors are independent between the experiments but are nevertheless correlated from one measurement to the next within each experiment. These uncertainties are reduced by more than the usual factor of √2. This can basically be seen as an effect of a cross-calibrating of the detectors with respect to each other using a large number of independent measurements.
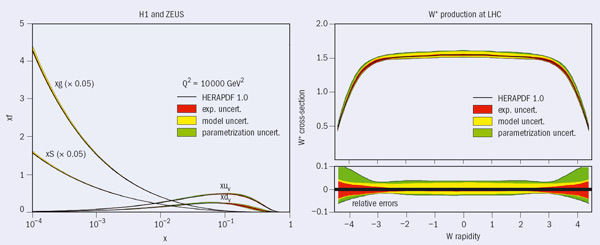
The paper on the measurement of inclusive deep inelastic scattering cross sections, submitted for publication by the H1 and ZEUS collaborations, contains a combination of more than 1402 individual measurements from 14 publications to obtain 741 cross-section measurements of unprecedented precision. All of the available data on neutral and charged-current interactions taken during the first phase of HERA running from 1992 to 2000 are used. The data cover virtualities, Q2, of the exchanged bosons from 0.2 GeV2 up to the highest values reachable at HERA of around 30,000 GeV2, and values of Bjorken-x as small as 0.2 × 10–6. These data extend into the electroweak regime from regions where perturbative QCD has never been tested. At small values of Bjorken-x, x <10–2, no other measurements exist. In this region the gain from the combination process is impressive: the individual measurements are dominated by systematic errors that become drastically reduced down to as little as 1%. These cross-sections depend on the universal proton-structure functions, F2, F3 andFL, which encapsulate the parton content of the proton. The structure function F2 dominates over most of the phase space, except at high Q2, where parity-violating weak effects lead to a non-zero contribution from xF3, and at large y, where the longitudinal part of the cross-section arising from gluon radiation leads by FL being sizeable.
Figure 1 shows parts of the universal structure function, F2, as a function of the variable x, for various values of the photon virtuality, Q2. The increase of F2 towards low x, discovered in the first years of HERA, is confirmed with a precision approaching 1%. As Q2 grows, F2 becomes steeper towards low x, reflecting the contributions to the quark component from gluon fluctuations in a qq pair. This rise is a fundamental discovery and reveals the role of gluons in binding nuclear matter. It is possible to decompose this structure function into one part that arises from “hard scattering” (so-called coefficient functions) and another non-perturbative part, which reflects the partonic content of the proton. Using the new data, the collaborations have extracted a new set of parton-distribution functions (HERAPDF 1.0), shown on the left in figure 2 for a high photon virtuality, Q2=10,000 GeV2. This partonic content is universal and can be used to make predictions for other processes involving protons – for example, cross-sections in proton–proton collisions at the LHC.
One example within the Standard Model is the production of single weak bosons at the LHC. This process can be regarded as a “standard candle” and can even be used to determine luminosity in the collider because the measurement can be done with great accuracy. The precision of the corresponding theoretical predications is dominated by the uncertainties that originate from the knowledge of the proton–parton distributions, which in turn come from the measurements at HERA (figure 2, right).
Ultimately, the H1 and ZEUS measurements provide the standard candle against which any new phenomenon at the LHC in the mass range of up to a few hundred giga-electron-volts will have to be compared. The new physics may well be in this range – in which case a precise knowledge of the production cross-section would be crucial in order to explore the properties of these new particles.
New physics arises in many theoretical extensions of the Standard Model. According to these extensions, a peak in a mass spectrum or a deviation in a certain variable should be observable. However, new physics can also manifest itself beyond the “standard predictions” and show up as spectacular events in regions of phase space where, according to the Standard Model, only few events should be seen. Events with energetic isolated leptons are an example of such a golden channel. Experimentally, they provide a clean signature; theoretically, they benefit from robust predictions.
The experiments at HERA reported the observation of events with isolated leptons (electrons or muons) and missing transverse momentum as early as 10 years ago. In the Standard Model this topology is explained by the production of a W boson, which decays to an energetic charged lepton and a neutrino. The neutrino escapes undetected, leading to “missing” momentum. The observation of such a rare process (typically one such event is recorded in 10 million other events) is a challenge and requires the full experimental information of the multilayer/multipurpose H1 and ZEUS detectors. Some of the observed events also contain a prominent hadronic jet – which makes them unlikely as W candidates because any hadronic recoil would typically be produced at low transverse momentum.
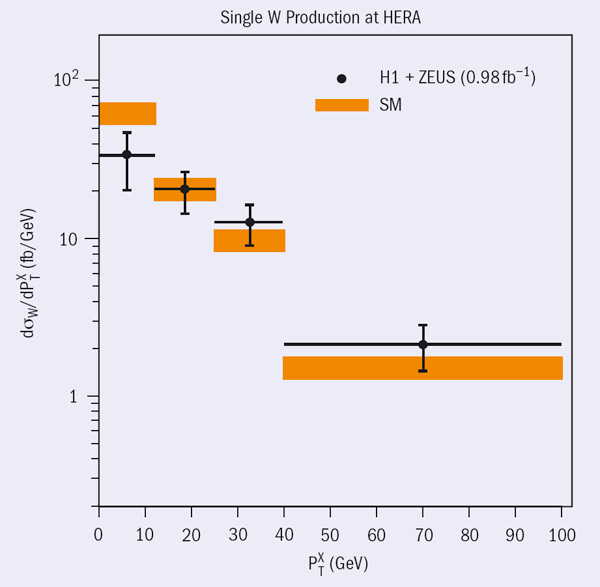
H1 observed a discrepancy with the Standard Model amounting to as much as three standard deviations, but with no effect seen in ZEUS. To clarify this point, the collaborations undertook a joint analysis effort. They investigated carefully all of the differences and studied all of the systematic effects. The individual results stand up to this scrutiny. By interpreting the difference as a statistical fluctuation, the two experiments can perform a common analysis. This leads to a decrease in the significance of the observed excess for events with large hadronic transverse momentum to below two standard deviations; it also improves significantly the measurement of the W cross-section (figure 3). Thus, this measurement becomes an important confirmation of the weak sector of the Standard Model in a unique configuration.
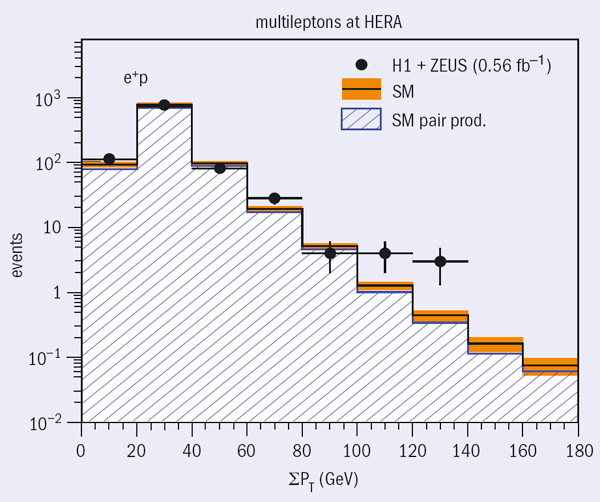
The third joint paper deals with events with more than one charged lepton that are dominantly produced by photon–photon collisions, the photons originating from the colliding electrons and protons. In individual analyses, H1 and ZEUS found a few hundred events containing several leptons, both electrons and muons, at high transverse momentum, including some events where the scalar sum of the lepton momenta exceeds 100 GeV. In a combined analysis, seven events are observed in this region in positron–proton collisions for an expected number of 1.9 ± 0.2, while no such event is observed in electron–proton collisions for a similar expectation (figure 4). The observation of the excess in positron–proton collisions is still compatible with the Standard Model and is interpreted as a statistical fluctuation. However, this observation stimulates discussion because it is also possible to attribute the excess to a bilepton resonance, such as a doubly charged Higgs boson, H++, produced in electroweak interactions.
These combined measurements from H1 and ZEUS are the first in a series of legacy results from the unique electron–proton collider, HERA. More than 20 years after the start of the facility and two years after the end of the data-taking, the harvest is in its best phase. This is also good for the LHC.