The Karlsruhe Tritium Neutrino (KATRIN) experiment has begun its seven-year-long programme to determine the absolute value of the neutrino mass.
On 11 June 2018, a tense silence filled the large lecture hall of the Karlsruhe Institute of Technology (KIT) in Germany. In front of an audience of more than 250 people, 15 red buttons were pressed simultaneously by a panel of senior figures including recent Nobel laureates Takaaki Kajita and Art McDonald. At the same time, operators in the control room of the Karlsruhe Tritium Neutrino (KATRIN) experiment lowered the retardation voltage of the apparatus so that the first beta electrons were able to pass into KATRIN’s giant spectrometer vessel. Great applause erupted when the first beta electrons hit the detector.
In the long history of measuring the tritium beta-decay spectrum to determine the neutrino mass, the ensuing weeks of KATRIN’s first data-taking opened a new chapter. Everything worked as expected, and KATRIN’s initial measurements have already propelled it into the top ranks of neutrino experiments. The aim of this ultra-high-precision beta-decay spectroscope, more than 15 years in the making, is to determine, by the mid-2020s, the absolute mass of the neutrino.
Massive discovery
Since the discovery of the oscillation of atmospheric neutrinos by the Super-Kamiokande experiment in 1998, and of the flavour transitions of solar neutrinos by the SNO experiment shortly afterwards, it was strongly implied that neutrino masses are not zero, but big enough to cause interference between distinct mass eigenstates as a neutrino wavepacket evolves in time. We know now that the three neutrino flavour states we observe in experiments – νe, νμ and ντ – are mixtures of three neutrino mass states.
Though not massless, neutrinos are exceedingly light. Previous experiments designed to directly measure the scale of neutrino masses in Mainz and Troitsk produced an upper limit of 2 eV for the neutrino mass – a factor 250,000 times smaller than the mass of the otherwise lightest massive elementary particle, the electron. Nevertheless, neutrino masses are extremely important for cosmology as well as for particle physics. They have a number density of around 336 cm–3, making them the most abundant particles in the universe besides photons, and therefore play a distinct role in the formation of cosmic structure. Comparing data from the Planck satellite together with data from galaxy surveys (baryonic acoustic oscillations) with simulations of the evolution of structure yields an upper limit on the sum of all three neutrino masses of 0.12 eV at 95% confidence within the framework of the standard Lambda cold-dark matter (ΛCDM) cosmological model.
Considerations of “naturalness” lead most theorists to speculate that the exceedingly tiny neutrino masses do not arise from standard Yukawa couplings to the Higgs boson, as per the other fermions, but are generated by a different mass mechanism. Since neutrinos are electrically neutral, they could be identical to their antiparticles, making them Majorana particles. Via the so-called seesaw mechanism, this interesting scenario would require a new and very high particle mass scale to balance the smallness of the neutrino masses, which would be unreachable with present accelerators.

As neutrino oscillations arise due to interference between mass eigenstates, neutrino-oscillation experiments are only able to determine splittings between the squares of the neutrino mass eigenstates. Three experimental avenues are currently being pursued to determine the neutrino mass. The most stringent upper limit is currently the model-dependent bound set by cosmological data, as already mentioned, which is valid within the ΛCDM model. A second approach is to search for neutrinoless double-beta decay, which allows a statement to be made about the size of the neutrino masses but presupposes the Majorana nature of neutrinos. The third approach – the one adopted by KATRIN – is the direct determination of the neutrino mass from the kinematics of a weak process such as beta decay, which is completely model-independent and depends only on the principle of energy and momentum conservation.

The direct determination of the neutrino mass relies on the precise measurement of the shape of the beta electron spectrum near the endpoint, which is governed by the available phase space (figure 1). This spectral shape is altered by the neutrino mass value: the smaller the mass, the smaller the spectral modification. One would expect to see three modifications, one for each neutrino mass eigenstate. However, due to the tiny neutrino mass differences, a weighted sum is observed. This “average electron neutrino mass” is formed by the incoherent sum of the squares of the three neutrino mass eigenstates, which contribute to the electron neutrino according to the PMNS neutrino-mixing matrix. The super-heavy hydrogen isotope tritium is ideal for this purpose because it combines a very low endpoint energy, Eo, of 18.6 keV and a short half-life of 12.3 years with a simple nuclear and atomic structure.
KATRIN is born
Around the turn of the millennium, motivated by the neutrino oscillation results, Ernst Otten of the University of Mainz and Vladimir Lobashev of INR Troitsk proposed a new, much more sensitive experiment to measure the neutrino mass from tritium beta decay. To this end, the best methods from the previous experiments in Mainz, Troitsk and Los Alamos were to be combined and upscaled by up to two orders of magnitude in size and precision. Together with new technologies and ideas, such as laser Raman spectroscopy or active background reduction methods, the apparatus would increase the sensitivity to the observable in beta decay (the square of the electron antineutrino mass) by a factor of 100, resulting in a neutrino-mass sensitivity of 0.2 eV. Accordingly, the entire experiment was designed to the limits of what was feasible and even beyond (see “Technology transfer delivers ultimate precision” box).
Technology transfer delivers ultimate precision
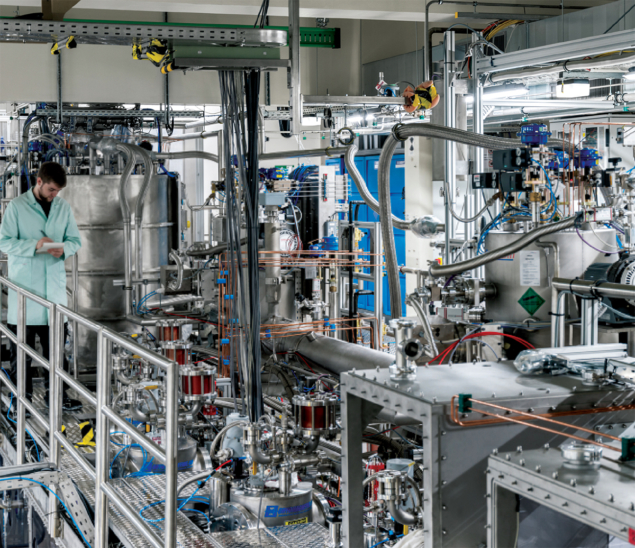
Many technologies had to be pushed to the limits of what was feasible or even beyond. KATRIN became a CERN-recognised experiment (RE14) in 2007 and the collaboration worked with CERN experts in many areas to achieve this. The KATRIN main spectrometer is the largest ultra-high vacuum vessel in the world, with a residual gas pressure in the range of 10–11 mbar – a pressure that is otherwise only found in large volumes inside the LHC ring – equivalent to the pressure recorded at the lunar surface.
Even though the inner surface was instrumented with a complex dual-layer wire electrode system for background suppression and electric-field shaping, this extreme vacuum was made possible by rigorous material selection and treatment in addition to non-evaporable getter technology developed at CERN. KATRIN’s almost 40 m-long chain of superconducting magnets with two large chicanes was put into operation with the help of former CERN experts, and a 223Ra source was produced at ISOLDE for background studies at KATRIN. A series of 83mKr conversion electron sources based on implanted 83Rb for calibration purposes was initially produced at ISOLDE. At present these are produced by KATRIN collaborators and further developed with regard to line stability.
Conversely, the KATRIN collaboration has returned its knowledge and methods to the community. For example, the ISOLDE high-voltage system was calibrated twice with the ppm-accuracy KATRIN voltage dividers, and the magnetic and electrical field calculation and tracking programme KASSIOPEIA developed by KATRIN was published as open source and has become the standard for low-energy precision experiments. The fast and precise laser Raman spectroscopy developed for KATRIN is also being applied to fusion technology.
KIT was soon identified as the best place for such an experiment, as it had the necessary experience and infrastructure with the Tritium Laboratory Karlsruhe. The KIT board of directors quickly took up this proposal and a small international working group started to develop the project. At a workshop at Bad Liebenzell in the Black Forest in January 2001, the project received so much international support that KIT, together with nearly all the groups from the previous neutrino-mass experiments, founded the KATRIN collaboration. Currently, the 150-strong KATRIN collaboration comprises 20 institutes from six countries.
It took almost 16 years from the first design to complete KATRIN, largely because many new technologies had to be developed, such as a novel concept to limit the temperature fluctuations of the huge tritium source to the mK scale at 30 K or the high-voltage stabilisation and calibration to the 10 mV scale at 18.6 kV. The experiment’s two most important and also most complex components are the gaseous, windowless molecular tritium source (WGTS) and the very large spectrometer. In the WGTS, tritium gas is introduced in the midpoint of the 10 m-long beam tube, where it flows out to both sides to be pumped out again by turbomolecular pumps. After being partially cleaned it is re-injected, yielding a closed tritium cycle. This results in an almost opaque column density with a total decay rate of 1011 per second. The beta electrons are guided adiabatically to a tandem of a pre- and a main spectrometer by superconducting magnets of up to 6 T. Along the way, differential and cryogenic pumping sections including geometric chicanes reduce the tritium flow by more than 14 orders of magnitude to keep the spectrometers free of tritium (figure 2).
Filtration

The KATRIN spectrometers operate as so-called MAC-E filters, whereby electrons are guided by two superconducting solenoids at either end and their momenta are collimated by the magnetic field gradient. This “magnetic bottle” effect transforms almost all kinetic energy into longitudinal energy, which is filtered by an electrostatic retardation potential so that only electrons with enough energy to overcome the barrier are able to pass through. The smaller pre-spectrometer blocks the low-energy part of the beta spectrum (which carries no information on the neutrino mass), while the 10 m-diameter main spectrometer provides a much sharper filter width due to its huge size.
The transmitted electrons are detected by a high-resolution segmented silicon detector. By varying the retarding potential of the main spectrometer, a narrow region of the beta spectrum of several tens of eV below the endpoint is scanned, where the imprint of a non-zero neutrino mass is maximal. Since the relative fraction of the tritium beta spectrum in the last 1 eV below the endpoints amounts to just 2 × 10–13, KATRIN demands a tritium source of the highest intensity. Of equal importance is the high precision needed to understand the measured beta spectrum. Therefore, KATRIN possesses a complex calibration and monitoring system to determine all systematics with the highest precision in situ, e.g. the source strength, the inelastic scattering of beta electrons in the tritium source, the retardation voltage and the work functions of the tritium source and the main spectrometer.
Start-up and beyond
After intense periods of commissioning during 2018, the tritium source activity was increased from its initial value of 0.5 GBq (which was used for the inauguration measurements) to 25 GBq (approximately 22% of nominal activity) in spring 2019. By April, the first KATRIN science run had begun and everything went like clockwork. The decisive source parameters – temperature, inlet pressure and tritium content – allowed excellent data to be taken, and the collaboration worked in several independent teams to analyse these data. The critical systematic uncertainties were determined both by Monte Carlo propagation and with the covariance-matrix method, and the analyses were also blinded so as not to generate bias. The excitement during the un-blinding process was huge within the KATRIN collaboration, which gathered for this special event, and relief spread when the result became known. The neutrino-mass square turned out to be compatible with zero within its uncertainty budget. The model fits the data very well (figure 3) and the fitted endpoint turned out to be compatible with the mass difference between 3He and tritium measured in Penning traps. The new results were presented at the international TAUP 2019 conference in Toyama, Japan, and have recently been published.
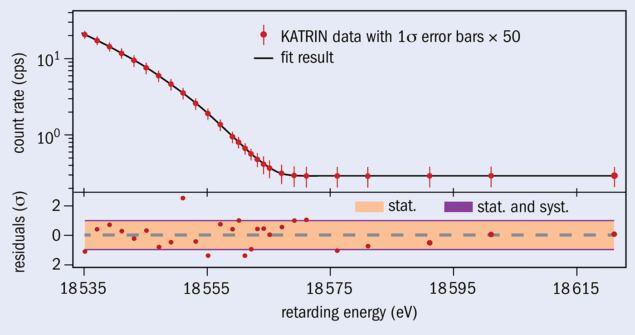
This first result shows that all aspects of the KATRIN experiment, from hardware to data-acquisition to analysis, works as expected. The statistical uncertainty of the first KATRIN result is already smaller by a factor of two compared to previous experiments and systematic uncertainties have gone down by a factor of six. A neutrino mass was not yet extracted with these first four weeks of data, but an upper limit for the neutrino mass of 1.1 eV (90% confidence) can be drawn, catapulting KATRIN directly to the top of the world of direct neutrino-mass experiments. In the mass region around 1 eV, the limit corresponds to the quasi-degenerated neutrino-mass range where the mass splittings implied by neutrino-oscillation experiments are negligible compared to the absolute masses.
The neutrino-mass result from KATRIN is complementary to results obtained from searches for neutrinoless double beta decay, which are sensitive to the “coherent sum” mββ of all neutrino mass eigenstates contributing to the electron neutrino. Apart from additional phases that can lead to possible cancellations in this sum, the values of the nuclear matrix elements that need to be calculated to connect the neutrino mass mββ with the observable (the half-life) still possess uncertainties of a factor two. Therefore, the result from a direct neutrino-mass determination is more closely connected to results from cosmological data, which give (model-dependent) access to the neutrino-mass sum.
A sizeable influence
Currently, KATRIN is taking more data and has already increased the source activity by a factor of four to close to its design value. The background rate is still a challenge. Various measures, such as out-baking and using liquid-nitrogen cooled baffles in front of the getter pumps, have already yielded a background reduction by a factor 10, and more will be implemented in the next few years. For the final KATRIN sensitivity of 0.2 eV (90% confidence) on the absolute neutrino-mass scale, a total of 1000 days of data are required. With this sensitivity KATRIN will either find the neutrino mass or will set a stringent upper limit. The former would confront standard cosmology, while the latter would exclude quasi-degenerate neutrino masses and a sizeable influence of neutrinos on the formation of structure in the universe. This will be augmented by searches for physics beyond the Standard Model, such as for sterile neutrino admixtures with masses from the eV to the keV scale.

Neutrino-oscillation results yield a lower limit for the effective electron-neutrino mass to manifest in direct neutrino-mass experiments of about 10 meV (50 meV) for normal (inverse) mass ordering. Therefore, many plans exist to cover this region in the future. At KATRIN, there is a strong R&D programme to upgrade the MAC-E filter principle from the current integral to a differential read-out, which will allow a factor-of-two improvement in sensitivity on the neutrino mass. New approaches to determine the absolute neutrino-mass scale are also being developed: Project 8, a radio-spectroscopy method to eventually be applied to an atomic tritium source; and the electron-capture experiments ECHo and HOLMES, which intend to deploy large arrays of cryogenic bolometers with the implanted isotope 163Ho. In parallel, the next generation of neutrinoless double beta decay experiments like LEGEND, CUPID or nEXO (as well as future xenon-based dark-matter experiments) aim to cover the full range of inverted neutrino-mass ordering. Finally, refined cosmological data should allow us to probe the same mass region (and beyond) within the next decades, while long-baseline neutrino-oscillation experiments, such as JUNO, DUNE and Hyper-Kamiokande, will probe the neutrino-mass ordering implemented in nature. As a result of this broad programme for the 2020s, the elusive neutrino should finally yield some of its secrets and inner properties beyond mixing.
Further reading
KATRIN Collaboration 2019 Phys. Rev. Lett. 123 221802.