Heavy-ion physics could be about to enter a new paradigm.

Image credit: F Ronchetti/CERN.
Heavy-ion and proton–proton collisions at ultrarelativistic energies provide a unique system with which to investigate the dynamics of matter in the early universe. By generating an incredibly hot and dense “fireball” of fundamental particles, such collisions allow us to recreate the extreme conditions of the universe during its first tens of microseconds of existence.
Given that the universe did not become transparent until roughly 370,000 years after the Big Bang, this epoch in our history lies completely out of reach to observational astronomy. According to the Standard Model of particle physics, the emergence of elementary particles and forces took place via a succession of symmetry-breaking mechanisms at different energy scales as the universe expanded and cooled. In the early universe, matter was made of freely roaming quarks – which formed the quark–gluon plasma (QGP) – in addition to leptons and gauge bosons. The QGP cooled down until hadrons including baryons such as neutrons and protons were formed. Photons continued interacting with charged particles until most of the matter became bound in neutral atoms, after which they were set free to form today’s cosmic microwave background.
During the past 30 years, a succession of collider experiments and impressive theoretical achievements have driven immense progress in the field of high-energy heavy-ion physics. Not only do these results shed new light on the dynamics of matter in the early universe, they probe fundamental predictions about the strong nuclear force governed by quantum chromodynamics (QCD).
Surprises galore
We have come a long way from the early belief in the 1970s that this early phase in the universe, recreated by colliding heavy ions at continuously increasing energies, comprised a gas of quarks and gluons. This is what was expected following asymptotic freedom, a feature of QCD that explains how the interaction between two quarks becomes asymptotically weaker as the distance between them decreases. But it took three major colliders on both sides of the Atlantic to find out what was really going on during these extreme initial moments.

Image credit: CERN EX-38-7-91.
The first big result came from CERN in 2000, when it was announced that heavy-ion collisions generated by the Super Proton Synchrotron (SPS) had created a new state of matter. CERN’s then Director-General, Luciano Maiani, worded the discovery as follows: From the combined data presented by the seven CERN experiments dedicated to the heavy-ion programme has emerged the clear picture that a new state of colour-deconfined matter has been created in the early stage of the collision that develops into a collective expansion of the fireball in the later stages.
This finding confirmed a fundamental prediction of QCD: above a critical temperature, quarks are no longer confined in hadrons. The CERN announcement was, however, only the beginning of our exploration into strongly interacting matter. The same year, the baton was passed to the Relativistic Heavy Ion Collider (RHIC) at Brookhaven National Laboratory in the US. Just five years after the CERN announcement, the remarkable data collected at RHIC demonstrated that a change of paradigm for strongly interacting matter was needed. The QGP that had been created in RHIC’s STAR and PHENIX experiments did not have the properties of a perfect gas. Rather, it showed all the properties of a perfect liquid: a strongly interacting fluid with minimal mean free path.
With RHIC continuing to produce data, in 2010 CERN rejoined the heavy-ion programme with the newly operational Large Hadron Collider (LHC) and the dedicated heavy-ion experiment ALICE, ATLAS, CMS and, more recently, LHCb. This machine marked a factor 25 jump in collision energy compared with RHIC, and its experiments confirmed with unprecedented precision the STAR and PHENIX findings. The LHC also offered new opportunities to explore deconfined matter in great detail, with the goal of understanding how the dynamics of matter emerge from the fundamental properties of the strong interaction and from the quark infrastructure of particles. More recently, and surprisingly, LHC data are pointing to unexpected similarities between observables measured in heavy-ion collisions and those measured in proton–lead or in high-multiplicity proton–proton collisions, perhaps hinting at yet another change of paradigm.
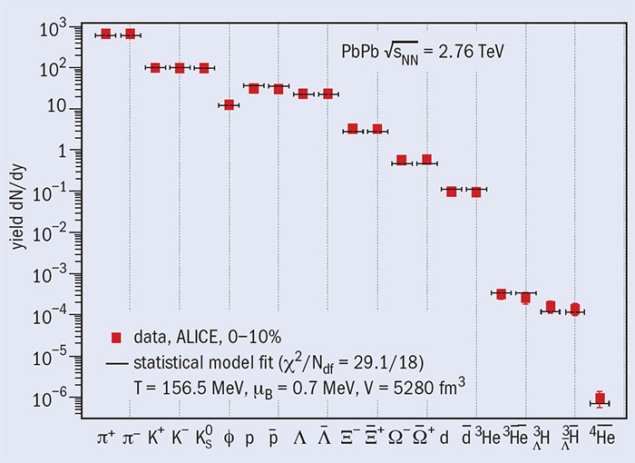
Image credit: arXiv:1611.01347.
The past 30 years have been an arduous path where every step both reveals more knowledge to us while simultaneously generating new riddles. To mark the important achievements so far and to discuss the long and thrilling future of heavy-ion physics, more than 400 physicists met at CERN on 9 November last year to review what can be considered as one of the most vigorous fields at the forefront of the high-energy physics programme.
A fitting celebration
Although accelerators had been working with electrons and protons for many decades, it was in 1974 when the Bevalac at Lawrence Berkeley Laboratory accelerated the first ions to relativistic energies (approximately 2 GeV per nucleon) and led to further programmes at BNL and CERN. The Bevalac beams were not energetic enough to create the necessary energy densities for the QGP to form, and it required the ingenuity of accelerator physicists and the remarkable development of electron cyclotron resonance (ECR) sources during the 1980s to take the decisive step toward “ultrarelativistic” energies.
The idea to launch an experimental heavy-ion programme at CERN came shortly after the SPS had enabled the discovery of the W and Z bosons in 1983. As the then CERN Director-General Herwig Schopper recalled at the November workshop, the 1980s were not the best time to initiate new projects. The CERN budget was severely cut and the laboratory was very much focused on the construction of the Large Electron–Positron Collider (LEP). Despite this, Schopper bravely decided to give heavy-ion physics a chance. He was motivated by arguments put forth by Reinhardt Stock, Hans Specht, Rudolf Bock, William Willis and several other leading physicists, but the main arguments that convinced him came from Tsung Dao Lee during a VIP lunch at CERN. Schopper recalled: “I knew [Lee] from the parity-violation experiment. He had no direct personal interest and his physics motivation sounded convincing. The main argument he put forward was to find the theoretically predicted quark–gluon plasma, which played an important role in the development of the universe.”
When, in October 1986, oxygen-16 ions were successfully accelerated by the SPS and fired into a fixed target of gold, the heavy-ion programme began with a disparate ensemble of detectors recuperated from earlier high-energy experiments. Six different experiments were hatched, each with a different profile adapted to hunt the variety of observables predicted to accompany the QGP phase transition: WA80 “Plastic Ball”; the NA34/2 HELIOS; the NA35 streamer chamber; NA36; WA85/94; and the NA38 muon-pair spectrometer.
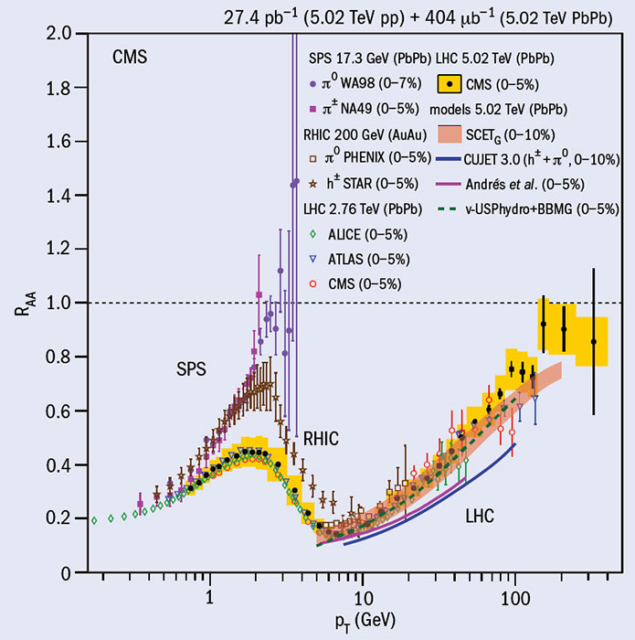
Image credit: arXiv:1611.01664.
In 1987, together with the increase of energy and the acceleration of sulphur beams, second-generation experiments containing innovative detector technologies were launched. Among these were: NA49 with an ambitious time projection chamber; CERES and its double ring imaging Cherenkov detectors; NA57 and its silicon tracking; and NA44, which contained a focusing spectrometer that made use of cesium-iodide photocathodes for the first time. The number of aficionados of this new and intriguing field of investigation grew rapidly from a few hundred initial physicists to the several thousand from all over the world who work on today’s LHC, SPS and RHIC heavy-ion facilities.
State of the art
Today, heavy-ion science is a thriving field of research, and it is notable that it is the common denominator in the physics programmes of all four major LHC experiments. On one hand, we have entered a phase of precision measurements of the QGP properties, while on the other hand the surprising similarities between proton–proton and proton–nucleon collisions observed at the LHC lead us to question if the same dynamics are at work in light and heavy systems. As demonstrated at the Quark Matter 2017 conference (see “Highlights from Quark Matter 2017” in Faces and Places), many new results are generating discussion. On the experimental side, these are based on a wealth of high-quality data collected both at LHC and RHIC for a variety of collision systems and energies, coupled with inventive analysis tools. On the theory side, particular progress has been made in relativistic hydrodynamics calculations. Among many new and creative theoretical concepts is the non-perturbative formulation of string theory, which along with the “AdS/CFT” correspondence provides tools to perform calculations for the QGP in the strongly coupled regime.
Macroscopic properties of the QGP such as its density and viscosity can now be determined with increasing precision by studying how the QGP, modelled by hydrodynamics, transports a perturbation. Measurements include the value of high-order flow coefficients and nonlinear mode mixing, while the value of η/S (shear viscosity over entropy density) and its temperature dependence have been pinned down within a factor two or less to 1/4π – which is the conjectured minimal value for a perfect quantum fluid.
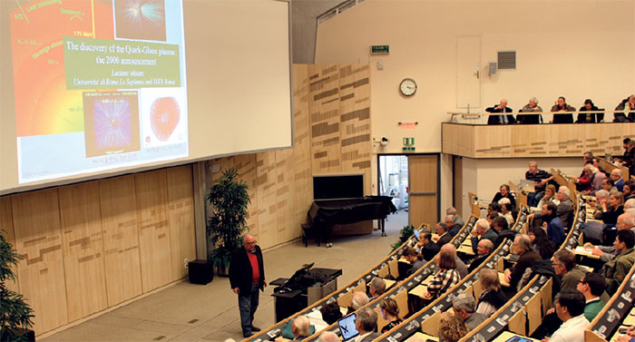
The microscopic structure of the QGP remains to be established, with the help of hard probes to provide the required resolving power. Here, jet quenching has already become a mundane phenomenon with which to study the content and dynamics of the QGP. In turn, the same studies also hint at the ability of the QGP to resolve the partonic shower. Quarkonia states, another hard probe, have also revealed rich dynamics. Their collision energy and transverse-momentum-dependent production can be understood in terms of two competing mechanisms: suppression due to resonance melting by colour screening and regeneration due to coalescence of free heavy-flavour quarks, both providing evidence for deconfinement. In addition, a flow signal has been measured for open and hidden charmed mesons, raising the question of whether charm quarks participate in the collective dynamics of the medium.
In general, the composition of the final hadronic state of the collision is quite well explained, assuming hadrons are formed in a thermalised state with a temperature that closely matches the temperature predicted for the QGP phase transition to the hadronic phase. Surprisingly, fragile objects such as light nuclei appear to be produced and to survive at temperatures several times larger than their binding energy. The possibility that nuclei were formed at the phase transition to hadrons, and not later via coalescence, would be an interesting complement to baryogenesis.
Strong future
As far as the next decades are concerned, in view of the achievements realised in the past years and the remaining open questions, it is a safe bet that heavy-ion physics will continue to be a vigorous field of research on both sides of the Atlantic. What are the relevant degrees of freedom of the QGP: perturbative partons, pseudo-particles, collective excitation of colour fields? Which dynamics drive the collision towards the formation of the QGP on timescales of a trillionth of a trillionth of a second, and in systems as small as a proton–proton collision? At which energy and which size does collectivity and statistical behaviour step in, and is chiral symmetry restored in the QGP?
These are some of the unanswered questions in the heavy-ion field. Existing and planned facilities that offer varying collision systems combined with ever more sophisticated detectors and strong collaborations between the theory and experiment communities are key to answering them. Based on what we are seeing currently, heavy-ion veteran Reinhard Stock commented that we could be about to enter a new paradigm with impact across high-energy physics. That would perhaps reveal QCD to be some sort of low-energy limit to a more fundamental theory.