Data from the LHC are providing input for models used to measure the highest-energy cosmic rays.
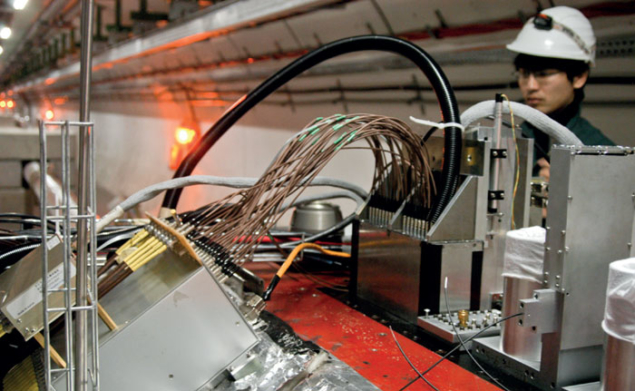
Recent observations of ultra-high-energy cosmic rays (UHECRs) by extensive air-shower arrays have revealed a clear cut-off in the energy spectrum at 1019.5 eV. The results are consistent with the predictions made in the mid-1960s that interactions with the cosmic microwave background would suppress the flux of particles at high energies (Greisen 1966, Zatsepin and Kuz’min 1966). Nevertheless, as the article on page 22 explains, the nature of the cut-off – and, indeed, the origin of the UHECRs – remains unknown.
UHECRs are observed in the large showers of particles created when a high-energy particle (proton or nucleus) interacts in the atmosphere. This means that information about the primary cosmic ray has to be estimated by “interpreting” the observed extensive air shower. Both longitudinal and lateral shower structures measured by the fluorescence and surface detectors, respectively, are used in the interpretation of the energy and species of the primary particle through comparison with the predictions of Monte Carlo simulations. In high-energy hadronic collisions, the energy flow is dominated by the very-forward-emitted particles in which the shower development is determined by the energy balance of baryonic and mesonic particle production. However, the lack of knowledge about hadronic interactions at such high energies, especially in the forward region, means that the interpretations tend to be model-dependent. To constrain the models used in the simulations, measurements of the forward production of particles relevant to air-shower development are indispensable at the highest energies possible.
Into the lab
The most important cross-section for cosmic-ray shower development is for the forward production in hadron collisions of neutral pions (π0), which immediately decay to two forward photons. The highest energies accessed in the laboratory are reached in particle colliders, and until the start-up of the LHC, the only experiment dedicated to forward particle production at a collider was carried out at UA7 at CERN’s SppS collider (Paré et al. 1990). Now, two decades later, members of the UA7 team have formed a new collaboration for the Large Hadron Collider forward (LHCf) experiment (LHCf 2006). This is dedicated to measuring very-forward particle production at the LHC, where running with proton–proton collisions at the full design energy of 14 TeV will correspond to 1017 eV in the laboratory frame and so will be in touch with the UHECR region.
The LHCf experiment consists of two independent calorimeters (Arm1 and Arm2) installed 140 m on either side of the interaction point in the ATLAS experiment. The detectors fit in the instrumentation slots of the target neutral absorbers (TANs), which are located where the vacuum chamber for the beam makes a Y-shaped transition from the single beam pipe that passes through the interaction point to the two separate beam tubes that continue into the arcs of the LHC. Charged particles produced in the collision region in the direction of the TAN are swept aside by an inner beam-separation magnet before they reach it. Consequently, only neutral particles produced at the interaction point enter the TAN and the detectors. This location allows the observation of particles at nearly 0° to the proton beam direction.
Both LHCf detectors contain two sampling and imaging calorimeters, each consisting of 44 radiation lengths of tungsten and 16 sampling layers of 3 mm-thick plastic scintillator for the initial runs. The calorimeters in Arm1 have an area transverse to the beam direction of 20 × 20 mm2 and 40 × 40 mm2, while those in Arm2 have areas of 25 × 25 mm2 and 32 × 32 mm2. Four X-Y layers of position-sensitive sensors are interleaved with the tungsten and scintillator to provide the transverse positions of the showers generated in the calorimeters, employing different technologies in the two detectors: Arm1 uses scintillating fibres and multi-anode photomultiplier tubes (MAPMTs); Arm2 uses silicon-strip sensors. In each case, the sensors are installed in pairs in such a way that two pairs are optimized to detect the maximum of gamma-ray-induced showers, while the other two are for hadronic showers developed deep within the calorimeters. Although the lateral dimensions of these calorimeters are small, the energy resolution is expected to be better than 6% and the position resolution better than 0.2 mm for gamma-rays with energy between 100 GeV and 7 TeV. This has been confirmed by test-beam results at CERN’s Super Proton Synchrotron.
LHCf successfully took data right from the first collision at the LHC in 2009 and finished its first phase of data-taking in mid-July 2010, after collecting enough data in proton–proton collisions at both 900 GeV and 7 TeV in the centre of mass. In 2011, the collaboration reported its measurements of inclusive photon spectra at 7 TeV (Adriani et al. 2011). A comparison of the data with predictions from the hadron-interaction models used in the study of air showers and from PYTHIA 8.145, which is popular in the high-energy-physics community, revealed various discrepancies, with none of the models showing perfect agreement with the data.
Now, LHCf has results for the inclusive π0 production rate at rapidities greater than 8.9 in proton–proton data at 7 TeV in the centre of mass. Using data collected in two runs in May 2010, corresponding to integrated luminosities of 2.53 nb–1 in Arm1 and 1.90 nb–1 in Arm2, the collaboration measured instances where two photons emitted into the very-forward regions could be attributed to π0 decays and obtained the transverse momentum (pT) distributions of the π0s. The criteria for the selection of π0 events were based on the position of the incident photons (within 2 mm of the edge of the calorimeter), the photon energy (above 100 GeV), the number of hits (one in each calorimeter), photon-like particle identification using the energy deposition and, last, an invariant mass corresponding to the π0 mass.
The pT spectra were derived in independent analyses of the two detectors, Arm1 and Arm2, in six rapidity intervals covering the range 8.9–11.0. These spectra, which agree within statistical and systematic errors, were then combined and compared with the predictions from various hadronic interaction models: DPMJET 3.04, QGSJET II-03, SIBYLL 2.1, EPOS 1.99 and PYTHIA 8.145 (default parameter set).

Figure 1 shows the combined spectrum for one rapidity interval, 9.2 < y < 9.4, compared with the outcome from these models (Adriani et al. 2012). It is clear that DPMJET 3.04 and PYTHIA 8.145 predict the π0 production rates to be higher than the data from LHCf as pT increases. SIBYLL 2.1 also predicts harder pion spectra than are observed in the experimental data, although the expected π0 yield is generally small. On the other hand, QGSJET II-03 predicts π0 spectra that are softer than both the LHCf data and the other model predictions. Among the hadronic interaction models, EPOS 1.99 shows the best overall agreement with the LHCf data.

In figure 2 the values of average pT (〈pT〉) obtained in this analysis are compared as a function of ylab = ybeam – y with the results from UA7 and with the model predictions. Although the LHCf and UA7 data have limited overlap and the systematic errors for UA7 are relatively large, the values of 〈pT〉 from the two experiments lie mainly along a common curve and there is no evidence of a dependence on collision energy. EPOS 1.99 shows the smallest dependence of 〈pT〉 on the two collision energies among three of the models, and this tendency is consistent with the results from LHCf and UA7. It is also evident from figure 2 that the best agreement with the LHCf data are obtained by EPOS 1.99.
The photon and π0 data from the LHCf experiment can now be used in models to constrain the mesonic part (or electromagnetic part via π0 s) of the air-shower development. The collaboration, meanwhile, is turning to analysis of baryon production, which will provide complementary information on the hadronic interaction. At the same time, work is ongoing towards taking data on proton–lead collisions at the LHC, planned for the end of 2012. Such nuclear collision data are important for understanding the interaction between cosmic rays and the atmosphere. Also other work is under way on replacing the plastic scintillator in the calorimeters – which were removed after the runs in July 2010 – with more radiation-resistant crystal scintillator, so as to be ready for 2014 when the LHC will run at 7 TeV per beam. There are also plans to change the position of the silicon sensors to improve the performance of the experiment in measuring the energy of the interacting particles.