An electron–positron collider to follow the LHC will produce copious Higgs bosons, yielding precise knowledge of this unique particle, explain Keith Ellis and Beate Heinemann.
Looking back on the great discoveries in particle physics, one can see two classes. The discovery of the Ω– in 1964 and of the top quark in 1995 were the final pieces of a puzzle – they completed an existing mathematical structure. In contrast, the discovery of CP violation in 1964 and of the J/ψ in 1974 opened up new vistas on the microscopic world. Paradoxically, although the Higgs boson was slated for discovery for almost half a century following the papers of Brout, Englert, Higgs, Weinberg and others, its discovery belongs in the second class. It constitutes a novel departure in the same way as the J/ψ and the discovery of CP violation, rather than the completion of a paradigm as represented by the discoveries of the Ω– and the top quark.
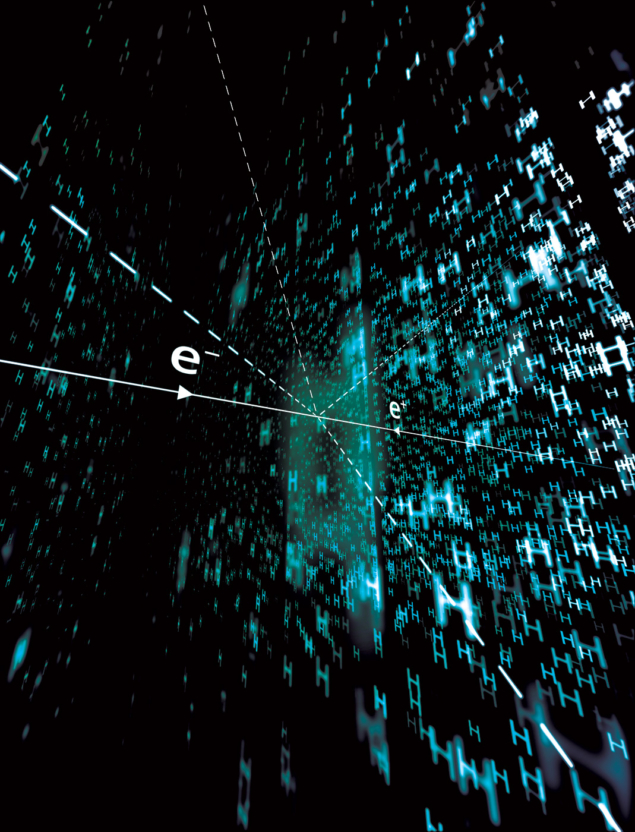
The novelty of the Higgs boson derives largely from its apparently scalar nature. It is the only fundamental particle without spin. Additionally, it is the only fundamental particle with a self-coupling (gluons also couple to other gluons, but only to those with different colour combinations). Measurements of the couplings of the Higgs boson to the W and Z bosons at the LHC have confirmed its role in the generation of their masses, likewise for the charged third-generation fermions. Despite this great success, the Higgs boson is connected to many of the most troublesome aspects of the Standard Model (see “Connecting the Higgs to Standard Model enigmas” panel). It is for this reason that the recently concluded update of the European strategy for particle physics advocated an electron–positron Higgs factory as the highest priority collider after the LHC, to allow detailed study of this novel and unique particle.
Circular vs linear
The discovery of the Higgs boson at the relatively light mass of 125 GeV, announced by the ATLAS and CMS collaborations in 2012, had two important consequences for experiment. The first was the large number of potentially observable branching fractions available. The second was that circular, as well as linear, e+e– machines could serve as Higgs factories. The two basic mechanisms for Higgs-boson production at such colliders are associated production, e+e– → ZH, and vector-boson fusion. The former process is dominant at the low-energy first stage of the various Higgs factories under consideration, with vector-boson fusion becoming more important with increasing energy (see “Channeling the Higgs” figure). About a quarter of a million Higgs bosons would be produced per inverse attobarn of data, leading to substantial numbers of recorded events even after the branching ratios to observable modes are taken into account.
Four Higgs-factory designs are presently being considered. Two are linear accelerators, namely the International Linear Collider (ILC) under consideration in Japan and the Compact Linear Collider (CLIC) at CERN, while the other two are circular: the Future Circular Collider (FCC-ee) at CERN and the Circular Electron Positron Collider (CEPC) in China.
The beams in circular colliders continuously lose energy due to synchrotron radiation, causing the luminosity at circular colliders to decrease with beam energy roughly as Eb–3.5. The advantage of circular colliders is their high instantaneous luminosity, in particular at the centre-of-mass energy relevant for the Higgs-physics programme (250 GeV), but even more so at lower energies such as those corresponding to the Z-boson mass (91 GeV). Electron and positron beams in a circular machine naturally achieve transverse polarisation, which can be exploited to make precise measurements of the beam energy via the electron and positron spin-precession frequencies.
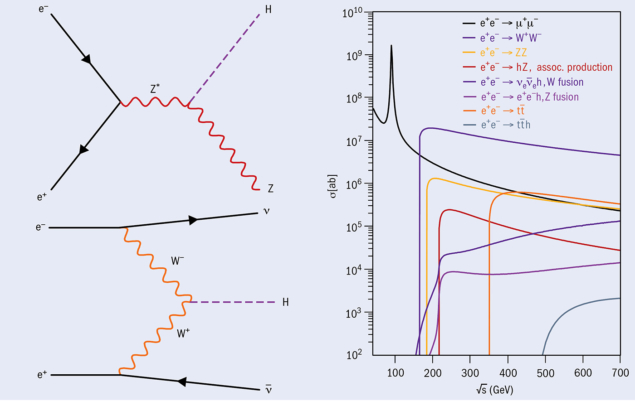
In contrast, for linear colliders the luminosity increases roughly linearly with the beam energy. The advantages of linear accelerators are that they can be extended to higher energies, and the beams can be polarised longitudinally. The ZH associated cross section can be increased by 40% with longitudinal polarisations of –80% and 30% for electrons and positrons, respectively. This increase, coupled with the ability to isolate certain components of Higgs-boson production by tuning the polarisation, enables a linear machine to achieve similar precisions on Higgs-boson measurements with half the integrated luminosity of a circular machine.
FCC-ee, CEPC and ILC are foreseen to run for several years at a centre-of-mass energy of around 250 GeV, where the ZH production cross section is largest. Instead, CLIC plans to run its first stage at 380 GeV where both WW fusion and ZH production contribute, and tt production is possible. The circular colliders FCC-ee and CEPC envisage running at the Z-pole and the WW production threshold for long enough to collect of the order 1012 Z bosons and 108 WW pairs, enabling powerful electroweak and flavour-physics programmes (see “Compare and contrast” table). To achieve design luminosity, all proposed e+e– colliders need beams focused to a very small size in one direction (30–70 nm for FCC-ee, 3–8 nm for ILC and 1–3 nm for CLIC), which are all below the values so far achieved at existing facilities.
Evolving designs
The proposed circular colliders are based on a combination of concepts that have been proven and used in previous and present colliders (LEP, SLC, PEP-II, KEKB, SuperKEKB, DAFNE). In Higgs-production mode the beam lifetime is limited by Bhabha scattering to about 30 minutes and therefore requires quasi-continuous injection or “top-up” as used by the B-factories. Each of the circular collider main concepts and parameters has been demonstrated in a previous machine, and thus the designs are considered mature. The total FCC-ee construction cost is estimated to be 10.5 billion CHF for energies up to 240 GeV, with an additional 1.1 billion CHF to go to the tt threshold. This includes 5.4 billion CHF for the tunnel, which could be reused later for a hadron collider. The CEPC cost has been estimated at $5 billion, including $1.3 billion for the tunnel. With the present design, the FCC-ee power consumption is 260–340 MW for the various energy stages (compared to 150 MW for the LHC).

The ILC was proposed in the late 1990s and a technical design report published in 2012. It uses superconducting RF cavities for the acceleration, as used in the currently operating European XFEL facility in Germany, to aim for gradients of 35 MV/m. The cost of the first energy stage (250 GeV) was estimated as $4.8–5.3 billion, with a power consumption of 130–200 MW, and an expression of interest to host the ILC as a global project is being considered in Japan. The CLIC accelerator uses a second beam, termed a drive-beam, to accelerate the primary beam, aiming for gradients in excess of 100 MV/m. This concept has been demonstrated with electron beams at the CLIC test facility, CTF3. The cost of the first energy stage of CLIC is estimated as 5.9 billion CHF with a power consumption of 170 MW, rising to 590 MW for final-stage operation at 3 TeV.
Another important difference between the proposed linear and circular colliders concerns the number of detectors they can host. Collisions at linear machines only occur at one interaction point, while in circular colliders at least two interaction points are proposed, doubling the luminosity available for analyses. Two detectors also offer the dual benefits of scientific competition and the cross-checking of results. At the ILC two detectors are proposed but they cannot run concurrently since they use the same interaction point.
FCC-ee and CLIC have both been proposed as CERN-hosted international projects, similar to the LHC or high-luminosity LHC (HL-LHC). At present, as recommended by the 2020 update of the European strategy for particle physics, a feasibility study for the FCC (including its post-FCC-ee hadron-collider stage, FCC-hh) is ongoing, with the goal of presenting an updated conceptual design report by the next strategy update in 2026. Among the e+e– colliders, CLIC has the greatest capacity to be extended to the multi-TeV energy range. In its low-energy incarnation it could be realised either with the drive-beam or conventional technology. CEPC is conceptually and technologically similar to FCC-ee and has also presented a conceptual design report. Nearly all statements about FCC-ee also hold for CEPC except that CEPC’s design luminosity is about a factor of two lower, and thus it takes longer to acquire the same integrated luminosity. At circular colliders, the multi-TeV regime (at least 100 TeV in the case of FCC-hh) would be reached by using proton beams, similar to what was done with LHC following LEP.
Connecting the Higgs to Standard Model enigmas

In addition to the vacuum expectation value of the Higgs field and the mass of the Higgs boson, the discovery of the Higgs boson introduces a large number of parameters into the Standard Model. Among them are the Yukawa couplings of the nine charged fermions (in contrast, the gauge sector of the SM has only three free parameters). The Yukawa forces, of which only three have been discovered corresponding to the couplings to the charged third-generation fermions, are completely new. They are of disparate strengths and, unlike the other forces, are not subject to the constraint of local gauge invariance. They provide a parameterisation of the theory of flavour, rather than an explanation. It is of primary importance to discover, bound and characterise the Yukawa forces. In particular, the discovery of CP violation in the Yukawa couplings would go beyond the confines of the Standard Model.
Famously, because of its scalar nature, the quantum corrections to the Higgs boson mass are only bounded by the cut-off on the theory, demanding large renormalisations to maintain the mass at 125 GeV as measured. This issue is not so much a problem for the Standard Model per se. However, in the context of a more complete theory that aims to supersede and encompass the Standard Model, it becomes much more troubling. In effect, the degree of cancellation necessary to maintain the Higgs mass at 125 GeV effectively sabotages the predictive power of any more complete theory. This sabotage becomes deadly as the scale of the new physics is pushed to higher and higher energies.
The electroweak potential is another area of importance in which our current knowledge is fragmentary. Within the confines of the Standard Model the potential is completely specified by the position of its minimum – the vacuum expectation value and the second derivative of the potential at the minimum, the mass of the Higgs boson (or equivalently its self-coupling). We have no direct knowledge of the behaviour of the potential at larger field values further from the minimum. In addition, extrapolation of the currently understood Higgs potential to higher energy reveals a world teetering between stability and instability. Further information about the behaviour of the potential could help us to interpret the meaning of this result. A modified electroweak potential might also give rise to a first-order phase transition at high temperature, rather than the smooth crossover expected for the Standard Model Higgs potential. This would fulfil one of the three Sakharov conditions necessary to generate an asymmetry between matter and antimatter in our universe.
To quantify the scientific reach of the proposed colliders compared to current knowledge or the expectations for the HL-LHC, it is necessary to define figures-of-merit for the observables that will be measured. For the Higgs boson the focus is on the coupling strengths to the Standard Model bosons and fermions, as well as the couplings to any new particles. The strength with which the Higgs boson couples to the various particles, i, is denoted by κi, defined such that κi = 1 corresponds to the Standard Model. Non-standard phenomena are included in this “kappa” framework by introducing two new quantities: the branching ratio into invisible particles (determined by measuring the missing energy in identified Higgs events), and the branching ratio to untagged particles (determined by measuring the contributions to the total width accounted for by the observed modes, or by directly searching for anomalous decays).
Higgs-boson observables
At hadron colliders, only ratios of κi parameters can be measured, since a precise measurement of the total width of the Higgs boson is lacking (the expected total width of the Higgs boson in the Standard Model is 4.2 MeV, which is far too small to be resolved experimentally). To determine the absolute κi values at a hadron collider a further assumption needs to be made, either on decay rates of the Higgs boson to new particles or on one of the κi values. An assumption that is often made, and valid in many beyond-the-Standard-Model theories, is that κZ ≤ 1.
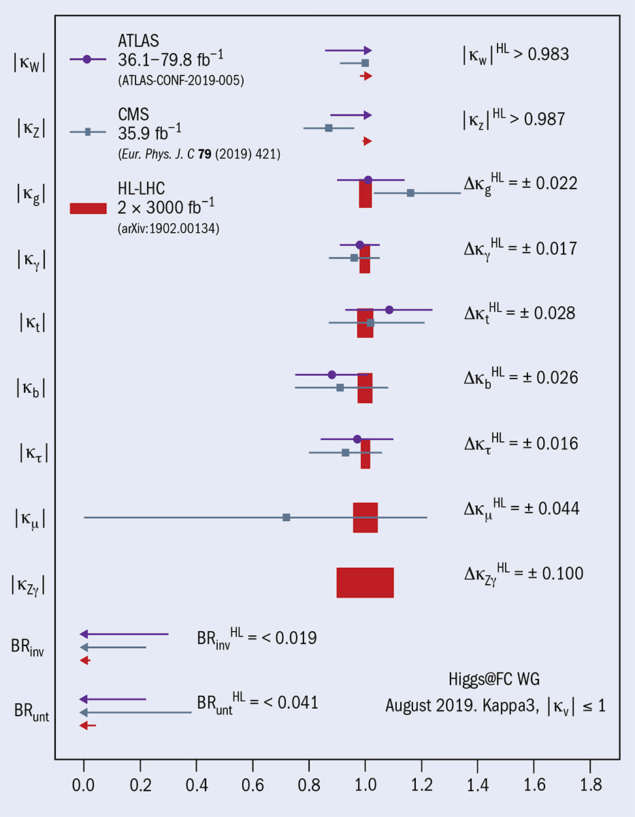
The kappa framework, however, by construction, does not parameterise possible effects coming from different Lorentz structures and/or the energy dependence of the Higgs couplings. Such effects could generically arise from the existence of new physics at higher scales and could lead not only to changes in the predicted rates, but also in distributions. Deviations of κi from 1 indicate a departure from the Standard Model, but do not provide a tool to diagnose its cause. This shortcoming is remedied in so-called effective-operator formalisms by including operators of mass dimension greater than four.
At e+e– colliders a Higgs boson produced via e+e– → ZH can be identified without observing its decay products. This measurement, of primary importance, is unique to e+e– colliders. By measuring the Z decay products and with the precise knowledge of the momenta of the incoming e– and e+ beams, the presence of the Higgs boson in ZH events can be inferred based on energy and momentum conservation alone, without actually tagging the Higgs boson. In this way one directly measures the coupling between the Higgs and Z bosons. In combination with the Higgs branching ratio to Z pairs it can be interpreted as a measurement of the Higgs-boson width. The first-stage e+e– Higgs factories all constrain the total width at about the 2% level.
LHC and HL-LHC
To assess the potential impact of the e+e– Higgs factories it is important to examine the point of departure provided by the LHC and HL-LHC. Since its startup in 2010 the LHC has made a monumental impact on our understanding of the Higgs sector. After the Higgs discovery in 2012, a measurement programme started and now, with nearly 150 fb–1 of data analysed by ATLAS and CMS, much has been learned. The Higgs-boson mass has been measured with a precision of < 0.2%, its spin and parity confirmed as expected in the Standard Model, and its coupling to bosons and to third-generation charged fermions established with a precision of 5–10%.
With the HL-LHC and its experiments planned to operate from 2027, the precision on the coupling parameters and the branching ratios to new particles will be increased by a factor of 5–10 in all cases, typically resulting in a sensitivity of a few % (see “Kappa couplings” figure). The HL-LHC will also enable measurements of the very rare μ+μ– decay, the first evidence for which was recently reported by CMS and ATLAS, and thus show whether the Higgs boson also generates the mass of a second-generation fermion. With the full HL-LHC dataset, corresponding to 3000 fb–1 for each of ATLAS and CMS, it is expected that di-Higgs production will be established with a significance of four standard deviations. This will allow a determination of the Higgs-boson’s coupling to itself with a precision of 50%.
About a quarter of a million Higgs bosons could be produced per inverse attobarn of data
The LHC has also made enormous progress in the direct searches for new particles at high energies. With more than 1000 papers published on this topic, hunting down particles predicted by dozens of theoretical ideas, and no firm sign of a new particle anywhere, it is clear that the new physics is either heavier, or more weakly coupled or has other features that hides it in the LHC data. The LHC is also a precision machine for electroweak physics, having measured the W-boson mass and the top-quark mass with uncertainties of 0.02% and 0.3%, respectively. In addition, a large number of relevant cross-section measurements of multi-boson production have been made, probing the trilinear and quartic interactions of the gauge bosons with each other.
Higgs-factory impact
In terms of the measurement precision on the Higgs-boson couplings, the proposed Higgs factories are expected to bring a major improvement with respect to HL-LHC in most cases (see “Relative precision” figure). Only for the rare decays to muons, photons and Zγ, and for the very massive top quark, is this not the case. The highest precision (0.2% in the case of FCC-ee) is achieved on κZ since the main Higgs production mode, ZH, depends directly on it, regardless of the decay mode. For other Standard Model particles, improvement factors of two to four are typical. For the invisible and untagged decays, the constraints are improved to around 0.2% and 1%, respectively, for some of the Higgs factories. A new measurement, not possible at the LHC, is that of the charm–quark coupling, κc.
None of the initial stages of the proposed Higgs factories will be able to directly probe the self-coupling of the Higgs boson beyond the 50% expected from the HL-LHC, since the cross-sections for the relevant processes (e+e– → ZHH and e+e– → HHνν) are negligible at centre-of-mass energies below 400 GeV. The Higgs self-coupling, however, enters through loops also in single-Higgs production and indirect effects might therefore be observable, for instance as a small (< 1%) deviation in measurements of the inclusive ZH cross section. Measurements of the Higgs self-coupling exploiting the di-Higgs production process can only be performed at higher energy colliders. The ILC and CLIC project uncertainties of around 30% at their intermediate energies and around 10% at their ultimate energies, while FCC-hh projects a precision of around 5%. Similarly, for the Higgs coupling to the top quark, the HL-LHC precision of 3.2% will not be improved by the initial stages of any of the Higgs factories.
The proposed Higgs factories also have a rich physics programme at lower energies, particularly at the Z pole. FCC-ee, for instance, plans to run for four years at the Z pole to accumulate a total of more than 1012 Z bosons – 100,000 times more than at LEP. This will enable a rich and unprecedented electroweak physics programme, constraining so-called oblique parameters (which are sensitive to violations of weak isospin) at the per-mille level, 100 times better than today. It will also enable a B-physics programme, complementary to that at Belle II and LHCb. At CEPC, a similar programme is possible, while at ILC and CLIC the luminosity when running at the Z pole is much lower: the typical number of Z-bosons that can be accumulated here is 109, 100 times more than LEP but not at the same level as the circular colliders. FCC-ee’s electroweak programme also foresees a run at the WW threshold to enable a high-precision measurement of the W mass.
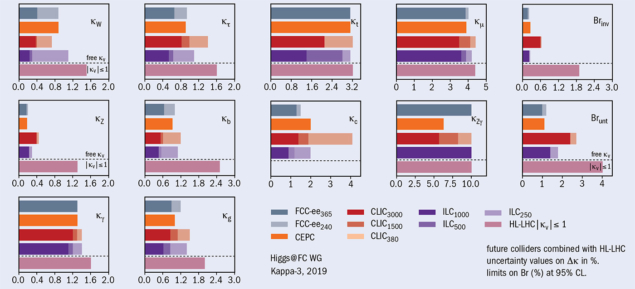
Concerning the large top-quark mass, measurements at the LHC suffer from uncertainties associated with renormalisation schemes and it is unlikely to improve the precision significantly at the HL-LHC beyond the currently achieved value of 400 MeV. At an e+e– collider operating at the tt threshold (~350 GeV), a measurement of the top mass with total uncertainty of around 50 MeV and with full control of the issues associated with the renormalisation scheme is possible. In addition to its importance as a fundamental parameter of the Standard Model, the top mass is the dominant term in the evolution of the Higgs potential with energy to determine vacuum stability (see “Connecting the Higgs to Standard Model enigmas” panel).
To assess the potential impact of the e+e– Higgs factories it is important to examine the point of departure provided by the LHC and HL-LHC
In short, a Higgs factory promises to expand our knowledge of nature at the smallest scales. The ZH cross-section measurement alone will probe fine tuning at a level of a few permille, about 30 times better than what we know today. This provides indirect sensitivity to new particles with masses up to 10–30 TeV, depending on their coupling strength, and could point to a new energy scale in nature.
But most of all the Higgs boson has not exhausted its ability to surprise. The rest of the Standard Model is a compact structure, exquisitely tested, and ruled by local gauge invariance and other symmetries. Compared to this, the Lagrangian of the Higgs sector is the wild west, where the final laws have yet to be written. Does the Higgs boson have a significant rate of invisible decays, which could be a key component in understanding the nature of dark matter in our universe? Does the Higgs boson act as a portal to other scalar degrees of freedom? Does the Higgs boson provide a source of CP violation? An electron–positron Higgs factory provides a tool to address these questions with unique clarity, when deviations between the measured and predicted values of observables are detected. Building on the data from the HL-LHC, it will be the perfect tool to elucidate the underlying laws of physics.
To explore all our coverage marking the 10th anniversary of the discovery of the Higgs boson ...
Further reading
A Abada et al. 2019 Eur. Phys. J. ST. 228 261.
P Bambade et al. 2019 arXiv:1903.01629.
J de Blas et al. 2020 JHEP 01 139 [arXiv:1905.03764].
P Burrows et al. 2018 arXiv:1812.06018.
R K Ellis et al. 2019 arXiv:1910.11775.
J B Guimaraes da Costa et al. 2018 arXiv:1811.10545.