Recent studies of exotic nuclides using traps and lasers at CERN’s ISOLDE facility are not only helping researchers understand nuclear structure, explain David Lunney and Gerda Neyens, but also offer new ways to look for physics beyond the Standard Model.

Understanding how the strong interaction binds the ingredients of atomic nuclei is the central quest of nuclear physics. Since the 1960s CERN’s ISOLDE facility has been at the forefront of this quest, producing the most extreme nuclear systems for examination of their basic characteristic properties.
A chemical element is defined by the number of protons in its nucleus, with the number of neutrons defining its isotopes. Apart from a few interesting exceptions, all elements in nature have at least one stable isotope. These form the so-called valley of stability in the nuclear chart of atomic number versus neutron number (see “Nuclear landscape” figure). Adding or removing neutrons disturbs the nuclear equilibrium and creates isotopes that are generally radioactive; the greater the proton–neutron imbalance, the faster the radioactive decay.
Most of the developments have been exported to other radioactive beam facilities around the world
The mass of a nucleus reveals its binding energy, which reflects the interplay of all forces at work within the nucleus from the strong, weak and electromagnetic interactions. Indications of sudden changes in the nuclear shape, when adding neutrons, are often revealed first indirectly as a sudden change in the mass, and can then be probed in detail by measurements of the charge radius and electromagnetic moments. Such diagnosis – performed by ion-trapping and laser-spectroscopy experiments on short-lived (from a few milliseconds upwards) isotopes – provides the first vital signs concerning the nature of nuclides with extreme proton-to-neutron ratios.
Recent mass-spectrometry measurements and high-precision measurements of nuclear moments and radii at ISOLDE demonstrate the rapid progress being made in understanding the stubborn mysteries of the nucleus. ISOLDE’s state-of-the-art laser-spectroscopy tools are also opening an era where molecular radioisotopes can be used as sensitive probes for physics beyond the Standard Model.
Tools of the trade
Progress in understanding the nucleus has gone hand in hand with the advancement of new techniques. Mass measurements of stable nuclei pioneered by Francis Aston nearly a century ago revealed a near-constant binding energy per nucleon. This pointed to a characteristic saturation of the nuclear force, which underlies the liquid-drop model and led to the semi-empirical mass formula for the nucleus developed by Bethe and von Weizsäcker. With the advent of particle accelerators in the 1930s, more isotopic mass data became available from reactions and decays, bringing new surprises. In particular, comparisons with the liquid drop revealed conspicuous peaks at certain so-called “magic” numbers (8, 20, 28, 50, 82, 126), analogous to the high atomic-ionisation potentials of the closed electron-shell noble-gas elements. These findings inspired the nuclear-shell model, developed by Maria Goeppert-Mayer and Hans Jensen, which is still used as an important benchmark today. The difference with the atomic system is that the force that governs the nuclear shells is poorly understood. This is because nucleons are themselves composite particles that interact through the complex interplay of three fundamental forces, rather than the single electromagnetic force governing atomic structure. The most important question in nuclear physics today is to describe these closed shells from fundamental principles (e.g. the strong interaction between quarks and gluons inside nucleons), to understand why shell structure erodes and how new shells arise far from stability.
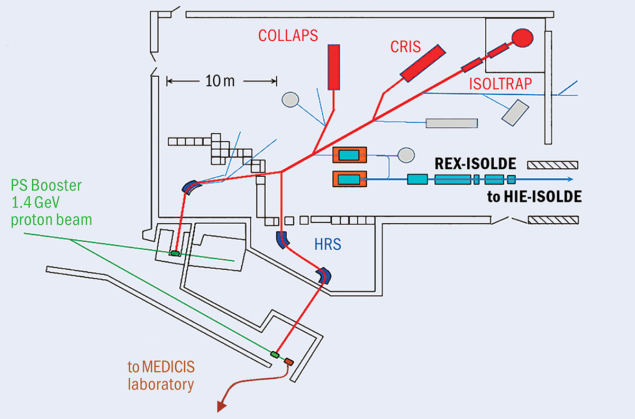
A key to reaching a deeper understanding of nuclear structure is the ability to measure the size and shape of nuclei. This was made possible using the precision technique of laser spectroscopy, which was pioneered with tremendous success at ISOLDE in the late 1970s. While increased binding energy is a tell-tale sign of a deforming nucleus, it gives no specific information concerning nuclear size or shape. Closed-shell configurations tend to favour spherical nuclei, but since these are rather rare, a particularly important feature of nuclei is their deformation. Inspecting electromagnetic moments derived from the measured atomic hyperfine structure and the change in charge radii derived from its isotopic shift provides detailed information about nuclear shapes and deformation, beautifully complementing mass measurements.
During the past half-century, nuclear science at ISOLDE has expanded beyond fundamental studies to applications involving radioactive tracers in materials (including biomaterials) and the fabrication of isotopes for medicine (with the MEDICIS facility). But the bulk of the ISOLDE physics programme, around 70%, is still devoted to the elucidation of nuclear structure and the properties of fundamental interactions. These studies are carried out through nuclear reactions, by decay spectroscopy, or by measuring the basic global properties – mass and size – of the most exotic species possible.
Half a century of history
The fabrication of extreme nuclear systems requires a driver accelerator of considerable energy, and CERN’s expertise here has been instrumental. After many years receiving proton beams from a 600 MeV synchrocyclotron (the SC, now a museum piece at CERN), ISOLDE now lies just off the beam line to the Proton Synchrotron (PS), receiving 1.4 GeV beam pulses from the PS Booster (see “ISOLDE from above” figure). ISOLDE in fact receives typically 50% of the pulses in the so-called super-cycle that links the intricate complex of CERN’s injectors for the LHC.
The heart of ISOLDE is a cylindrical target that can contain various different materials. The stable nuclei in the target are dissociated by the proton impact and form exotic combinations of protons and neutrons. Heating the target (up to 2000 degrees) helps these fleeting nuclides to escape into an ionisation chamber, in which they form 1+ ions that are electrostatically accelerated to around 50 keV. Isotopes of one particular mass are selected using one of two available mass separators, and subsequently delivered to the experiments through more than a dozen beamlines. A similar number of permanent experimental setups are operated by several small international collaborations. Each year, more than 40 experiments are performed at ISOLDE by more than 500 users. More than 900 users from 26 European and 17 non-European countries around the world are registered as members of the ISOLDE collaboration.
A new era for fundamental physics research has opened up
ISOLDE sets the global standard for the production of exotic nuclear species at low energies, producing beams that are particularly amenable to study using precision lasers and traps developed for atomic physics. Hence, ISOLDE is complementary to higher energy, heavy-ion facilities such as the Radioactive Isotope Beam Factory (RIBF) at RIKEN in Japan, the future Facility for Rare Isotope Beams (FRIB) in the US, and the Facility for Antiproton and Ion Research (FAIR/GSI) in Europe. These installations produce even more exotic nuclides by fragmenting heavy GeV projectiles on a thin target, and are more suitable for studying high-energy reactions such as breakup and knock-out. Since 2001, ISOLDE has also driven low-energy nuclear-reaction studies by installing a post-accelerator that enables exotic nuclides to be delivered at MeV energies for the study of more subtle nuclear reactions, such as Coulomb excitation and transfer. Post-accelerated radioactive beams have superior optical quality compared to the GeV beams from fragment separators so that the radioactive beams accelerated in the REX and more recent HIE-ISOLDE superconducting linacs enable tailored reactions to reveal novel aspects of nuclear structure.

ISOLDE’s state-of-the art experimental facilities have evolved from more than 50 years of innovation from a dedicated and close-knit community, which is continuously expanding and also includes material scientists and biochemists. The pioneering experiments concerning binding energies, charge radii and moments were all performed at CERN during the 1970s. This work, spearheaded by the Orsay group of the late Robert Klapisch, saw the first use of on-line mass separation for the identification of many new exotic species, such as 31Na. This particular success led to the first precision mass measurements in 1975 that hinted at the surprising disappearance of the N = 20 shell closure, eight neutrons heavier than the stable nucleus 23Na. In collaboration with atomic physicists at Orsay, Klapisch’s team also performed the first laser spectroscopy of 31Na in 1978, revealing the unexpected large size of this exotic isotope. To reach heavier nuclides, a mass spectrometer with higher resolution was required, so the work naturally continued at the expanding ISOLDE facility in the early 1980s.
Meanwhile, another pioneering experiment was initiated by the group of the late Ernst-Wilhelm Otten. After having developed the use of optical pumping with spectral lamps in Mainz to measure charge radii, Otten’s group exploited ISOLDE’s first offerings of neutron-deficient Hg isotopes and discovered the unique feature of shape-staggering in 1972. Through continued technical improvements, the Mainz group established the collinear laser spectroscopy (COLLAPS) programme at ISOLDE in 1979, with results on barium and ytterbium isotopes. When tunable lasers and ion traps became available in the early 1980s, the era of high-precision measurements of radii and masses began. These atomic-physics inventions have revolutionised the study of isotopes far from stability and the initial experimental set-ups are still in use today thanks to continuous upgrades and the introduction of new measurement methods. Most of these developments have been exported to other radioactive beam facilities around the world.
Mass measurements with ISOLTRAP
ISOLTRAP is one of the longest established experiments at ISOLDE. Installed in 1985 by the group of Hans-Jürgen Kluge from Mainz, it was the first Penning trap on-line at a radioactive beam facility, spawning a new era of mass spectrometry. The mass is determined from the cyclotron frequency of the trapped ion, and bringing the technique on line required significant and continuous development, notably with buffer-gas cooling techniques for ion manipulation. Today, ISOLTRAP is composed of four ion traps, each of which has a specific function for preparing the ion of interest to be weighed.
Since the first results on caesium, published in 1987, ISOLTRAP has measured the masses of more than 500 species spanning the entire nuclear chart. The most recent results, published this year by Vladimir Manea (Paris-Saclay), Jonas Karthein (Heidelberg) and colleagues, concern the strength of the N = 82 shell closure below the magic (Z = 50) 132Sn from the masses of (Z = 48) 132,130Cd. The team found that the binding energy only two protons below the closed shell was much less than what was predicted by global microscopic models, stimulating new ab-initio calculations based on a nucleon–nucleon interaction derived from QCD through chiral effective-field theory. These calculations were previously available for lighter systems but are now, for the first time, feasible in the region just south-east of 132Sn, which is of particular interest for the rapid neutron-capture process creating elements in merging neutron stars.
The other iconic doubly magic nucleus 78Ni (Z = 28, N = 50) is not yet available at ISOLDE due to the refractory nature of nickel, which slows its release from the thick target so that it decays on the way out. However, the production of copper – just one proton above – is so good that CERN’s Andree Welker and his colleagues at ISOLTRAP were recently able to probe the N = 50 shell by measuring the mass of its nuclear neighbour 79Cu, finding it to be consistent with that of the doubly magic 78Ni nucleus. Masses from large-scale shell-model calculations were in excellent agreement with the observed copper masses, indicating the preservation of the N = 50 shell strength but with some deformation energy creeping in to help. Complementary observables from laser spectroscopy helped to tell the full story, with results on moments and radii from the COLLAPS and the more recent Collinear Resonance Ionization Spectroscopy (CRIS) experiments adding an interesting twist.
Laser spectroscopy with COLLAPS and CRIS
Quantum electrodynamics provides its predictions of atomic energy levels mostly by assuming the nucleus is point-like and infinitely heavy. However, the nucleus indeed has a finite mass as well as non-zero charge and current distributions, which impact the fine structure. Thus, complementary to the high-energy scattering experiments used to probe nuclear sizes, the energy levels of orbiting electrons offer a marvellous probe of the electric and magnetic properties of the nucleus. This fact is exploited by the elegant technique of laser spectroscopy, a fruitful marriage of atomic and nuclear physics realised by the COLLAPS collaboration since the late 1970s. COLLAPS uses tunable continuous-wave lasers for high-precision studies of exotic nuclear radii and moments, and similar setups are now running at other facilities, such as Jyvaskyla in Finland, TRIUMF in Canada and NSCL-MSU in the US.
A recent highlight from COLLAPS, obtained this year by Simon Kaufmann of TU Darmstadt and co-workers, is the measurement of the charge radius of the exotic, semi-magic isotope 68Ni. Such medium-mass exotic nuclei are now in reach of the modern ab-initio chiral effective-field theories, which reveal a strong correlation between the nuclear charge radius and its dipole polarisability. With both measured for 68Ni, the data provide a stringent benchmark for theory, and allow researchers to constrain the point-neutron radius and the neutron skin of 68Ni. The latter, in turn, is related to the nuclear equation-of-state, which plays a key role in supernova explosions and compact-object mergers, such as the recent neutron-star merger GW170817.

Building on pioneering work by COLLAPS, the collinear laser beamline, CRIS, was constructed at ISOLDE 10 years ago by a collaboration between the groups of Manchester and KU Leuven. In CRIS, a bunched atom beam is overlapped with two or three pulsed laser beams that are resonantly laser-ionised via a particular hyperfine transition. These ions are then deflected from the remaining background atoms and counted in quasi background-free conditions. CRIS has dramatically improved the sensitivity of the collinear laser spectroscopy method so that beams containing just a few tens of ions per second can now be studied with the same resolution as the optical technique of COLLAPS.
Ruben de Groote of KU Leuven and co-workers recently used CRIS to study the moments and charge radii of the copper isotopes up to 78Cu, providing critical information on the wave function and shape of these exotic neighbours, and insight on the doubly magic nature of 78Ni. Both the ISOLTRAP and CRIS results provide a consistent picture of fragile equilibrium in 78Ni, where the failing strength of the proton and neutron shell closures is shored up with binding energy brought by slight deformation.
These precision measurements in new regions of the nuclear chart bring complementary observables that must be coherently described by global theoretical approaches. They have stimulated and guided the development of new ab-initio results, which now allow the properties of extreme nuclear matter to be predicted. While ISOLDE cannot produce absolutely all nuclides on the chart (for example, the super-heavy elements), precision tests in other, key regions provide confidence in the global-model predictions in regions unreachable by experiment.
Searches for new physics
By combining the ISOLDE expertise in radioisotope production with the mass spectrometry feats of ISOLTRAP and the laser spectroscopy prowess from the CRIS and RILIS (Resonant Ionization Laser Ion Source) teams, a new era for fundamental physics research has opened up. It is centred on the ability of ISOLDE to produce short-lived radioactive molecules composed of heavy pear-shaped nuclei, in which a putative electric dipole moment (EDM) would be amplified to offer a sensitive test of time-reversal and other fundamental symmetries. Molecules of radium fluoride (RaF) are predicted to be the most sensitive probes for such precision studies: the heavy mass and octupole-deformed (pear shape) of some radium isotopes, immersed in the large electric field induced by the molecular RaF environment, makes these molecules very sensitive probes for symmetry-violation effects, such as the existence of an EDM. However, these precision studies require laser cooling of the RaF molecules, and since all isotopes of Ra are radioactive, the molecular spectroscopy of RaF was only known theoretically.
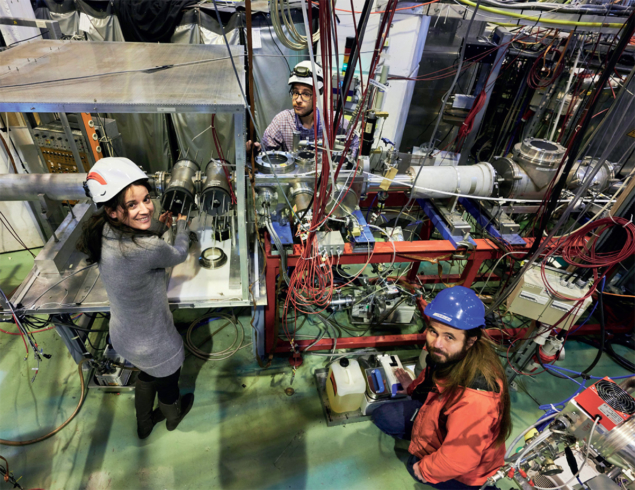
This year, for the very first time, an ISOLDE collaboration led by CRIS collaborator Ronald Garcia Ruiz at CERN was able to produce, identify and study the spectroscopy of RaF molecules, containing different long-lived radioisotopes of radium. Specific Ra isotopes were chosen because of their octupole nature, as revealed by experiments at the REX- and HIE-ISOLDE accelerators in 2013 and 2020. The measured molecular excitation spectral properties provide clear evidence for an efficient laser-cooling scheme, providing the first step towards precision studies.
Many interesting new-physics opportunities will open up using different kinds of radioactive molecules tuned for sensitivity to specific symmetry violation aspects to test the Standard Model, but also with potential impact in nuclear physics (for example, enhanced sensitivity to specific moments), chemistry and astrophysics. This will also require dedicated experimental set-ups, combining lasers with traps. The CRIS collaboration is preparing these new set-ups, and the ability to produce RaF and other radioactive molecules is also under investigation at other facilities, including TRIUMF and the low-energy branch at FRIB. More than 50 years after its breakthrough beginning, ISOLDE continues to forge new paths both in applied and fundamental research.
Further reading
R P de Groote et al. 2020 Nat. Phys. 16 620 and 2017 Phys. Rev. C 96 041302(R).
R F Garcia Ruiz et al. 2020 Nature 581 396.
S Kaufmann et al. 2020 Phys. Rev. Lett. 124 132502.
D Lunney (on behalf of the ISOLTRAP Collaboration) 2017 J. Phys. G 44 064008.
V Manea et al. 2020 Phys. Rev. Lett. 124 092502.
R Neugart et al. (on behalf of COLLAPS and CRIS Collaborations) 2017 J. Phys. G 44 064002.
A Welker et al. 2017 Phys. Rev. Lett. 119 192502.