With 9 T dipole magnets of a new “twin” design and superfluid helium cooling, the LHC took the use of superconductivity in accelerators to a new level.

As the 1970s turned into the 1980s, two projects at the technology frontier were battling it out in the US accelerator community: the Energy Doubler, based on Robert Wilson’s vision to double the energy of the Main Ring collider at Fermilab; and Isabelle (later the Colliding Beam Accelerator) in Brookhaven. The latter was put in question by the difficulty in increasing the magnetic field from 4 T to 5 T – which turned out to be much harder than originally thought – and eventually gave way to Carlo Rubbia’s idea to transform CERN’s Super Proton Synchrotron into a p–p collider, allowing the first detection of W and Z particles. Fermilab’s project, however, became a reality. Based on 800 superconducting dipole magnets with a field in excess of 4 T, it involved the first ever mass-production of superconductor and represented a real breakthrough in accelerator technology. For the first time, a circular accelerator had been built to work at a higher energy without increasing its radius.
When the Tevatron began operation at 540 GeV in 1983, Europe was just starting to build HERA at DESY. This electron–proton collider included a 6 km ring of superconducting magnets for the 820 GeV protons and it came into operation in 1989. The 5 T dipoles for HERA were the first to feature cold iron and – unlike the Tevatron magnets, which were built in house – they were produced by external companies, thus marking the industrialization of superconductivity.
Meanwhile the USSR was striving to build a 3 TeV superconducting proton synchrotron (UNK), which was later halted by the collapse of the Soviet Union, while at CERN the idea was emerging to build a Large Hadron Collider in the tunnel constructed for the Large Electron–Positron (LEP) collider (CERN Courier October 2008 p9). However, the US raised the bid with a study for the “definitive machine”. The Superconducting Super Collider (SSC), which was strongly supported by the US Department of Energy and by President Reagan, would accelerate two proton beams to 20 TeV in a ring of 87 km circumference with 6.6 T superconducting dipoles. With this size and magnetic field, the SSC would require decisive advances in superconductors as well as in other technologies. When the then director-general of CERN, Herwig Schopper, attended a high-level official meeting in the US and asked what influence on the scientific and technical goals the Europeans could have by joining the project, he was told “none, either you join the project as it is or you are out”. This ended the possibility of collaboration and the competition began.
To compete with the SSC, the LHC had to fight on two fronts: increase the magnetic field as much as possible so as to reduce the handicap of the relatively small circumference of the LEP tunnel; and increase the luminosity as much as possible to compensate for the inevitable lower energy. In addition, CERN had to cope with a tunnel with a cross-section that was tiny for a hadron collider, which many considered a “poisoned gift” from LEP. However, the interest for young physicists and engineers lay in the “impossible challenges” that the LHC presented.
To begin with, there was the 8–10 T field in a dipole magnet. Such a large step with respect to the Tevatron would require both the use of large superconducting cable to carry 13 kA in operating conditions of 10 T – almost double the capability of existing technology – and cooling by superfluid helium at 1.8–1.9 K. Never previously used in accelerators, superfluid helium cooling had been developed for TORE Supra, the tokamak project led by Robert Aymar but on a smaller scale. Then, to fit the exiting LEP tunnel, the magnets would have to be of an innovative “two-in-one” design – first proposed by Brookhaven but discarded by US colleagues for the SSC – where two magnetic channels are hosted in the iron yoke within a single cold mass and cryostat. In this way, a 1 m diameter cryostat could house two magnets, while the geometry of the SSC (with separate magnets but with 30% lower field than the LHC) simply could not fit in the LHC tunnel. Figure 1 shows the various main-dipole cross-sections for the various hadron machines.
A critical milestone
In 1986 R&D on the LHC started under the leadership of Giorgio Brianti, quietly addressing the three issues specific to the LHC (high field, superfluid helium and two-in-one), while relying on the development done for HERA and especially for the SSC for almost all of the other items that needed to be improved. The high field was the critical issue and had to be tested immediately. Led by Romeo Perin and Daniel Leroy, CERN produced the first LHC coil layout and provided the first large superconducting cable to Ansaldo Componenti. This company then manufactured on its own a 1-m long dipole model – single bore, without a cryostat – that was tested at CERN in 1987. Reaching a field of 9 T at 1.8 K, it proved the possibility of reaching the region of 8–10 T (CERN Courier October 2008 p19). This was arguably the most critical milestone of the project because it gave credibility to the whole plan and began to lay doubt on the strategy for the SSC.

These results were obtained with niobium-titanium alloy (Nb-Ti), the workhorse of superconductivity. CERN had also a parallel development with niobium-tin (Nb3Sn) that could have produced a slightly higher field at 4.5 K, with standard helium cooling. This development, pursued with the Austrian company Elin and led by CERN’s Fred Asner, produced a 1-m long 9.8 T magnet and also a 10.1 T coil in mirror configuration, the first accelerator coil to break the 10 T wall. However, in 1990 the development work on Nb3Sn was stopped in favour of the much more advanced and practical Nb-Ti operating at 1.9 K. This was a difficult decision, as Nb3Sn had a greater potential than Nb-Ti and would avoid the difficulty of using superfluid helium, but it was vitally important to concentrate resources and to have a viable project in a short time. The decision was similar that taken by John Adams in the mid-1970s to abandon the emerging superconducting technology in favour of more robust resistive magnets for CERN’s Super Proton Synchrotron.
For the development of the superconducting cable there were three main issues. First, it should reach a sufficient critical current density with a uniformity of 5–10% over the whole production, which also had to be guaranteed in the ratio between the superconductor and the stabilizing copper matrix, illustrated in figure 2. The critical current was to be optimized at 11 T at 1.9 K, maximizing the gain when passing from 4.2 to 1.9 K. The second issue was to reduce the size of the superconducting filaments to 5 μm without compromising the critical current. This required, among other features, the development of a niobium barrier around Nb-Ti ingots. Third was to control the dynamic (ramping up) effect in a large cable, as some effects vary as the cube of the width.
Again, the strategy was to concentrate on specific LHC issues – the large cable, the critical current optimization at 1.9 K – and rely on the SSC’s more advanced development for the other issues. There is, indeed, a large debt that CERN owes to the SSC project for the superconductor development. However, when the SSC project was cancelled in 1993, the problem of eliminating the dynamic effect arising from the resistance between strands composing the cable was still unresolved – but it became urgent in view of the results on the first long magnets in 1994 and after. Later, CERN carried out intense R&D to find a solution suitable for mass production relatively late, at the end of the 1990s. This involved controlled oxidation, after cable formation, of the thin layer of tin-silver alloy with which all the copper/Nb-Ti strands were coated – a technology that was a step beyond the SSC development.
Returning to the magnet development, after the success of the 1987 model magnet, which was replicated by another single-bore magnet that CERN ordered from Ansaldo, the R&D route split into two branches. One concerned the manufacture of 1-m long full-field LHC dipole magnets to prove the concept of high fields in the two-in-one design, with superconducting cable and coil geometry close to the final ones. A few bare magnets, called Magnet Twin Aperture (MTA), were commissioned from European Industry (Ansaldo, Jeumont-Scheinder consortium, Elin, Holec) under the supervision of Leroy at CERN.
The second line of development lay in proving the two-in-one concept in long magnets and a superfluid-helium cryostat. This involved assembling superconducting coils from the HERA dipole production, which had ended in 1988, in a single cold mass and cryostat, the Twin Aperture Prototype (TAP). The magnet, under the supervision of Jos Vlogaert with the cryostat and cold-mass, under Philippe Lebrun, tested successfully in 1990, reaching 5.7 T at 4.2 K and 7.3–8.2 T at 1.8 K – thus supporting the choices of the two-in-one magnet design, of the superfluid helium cooling and the new cryostat design.
At the same time, the LHC dipole was designed in the years 1987–1990, featuring an extreme variation: the “twin” concept, where the two coil apertures are fully coupled, i.e. with no iron between the two magnetic channels (figure 3). We now take this design for granted, but at the time there was scepticism within the community (especially across the Atlantic); it was supposed to be much more vulnerable to perturbations because of the coupling and to present an irresolvable issue with field quality. It is to the great credit of the CERN management and especially Perin, who for a long time was head of the magnet group, that they defended this design with great resilience – because among many advantages it also made an important 15% saving in the cost.
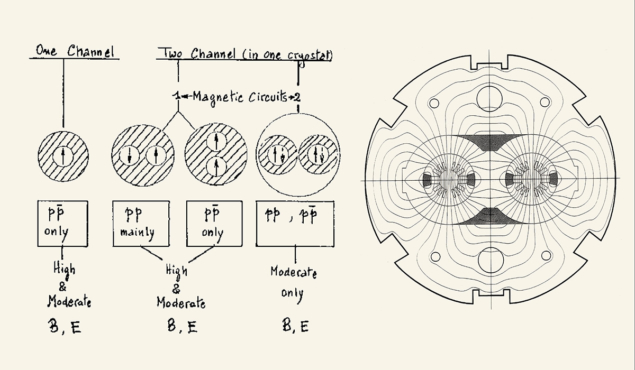
The result of the first sets of twin 1-m long magnets came in 1991–1992. Some people were disappointed because they felt that the results fell short of the 10 T field “promised” in the LHC “pink book” of 1990. However, anyone who knows superconductivity greatly appreciated that the first generation of twins went well over 9 T. This was already a high field and only 5–10% less than expected from the so-called “short sample” (the theoretical maximum inferred by measuring the properties of a short 50–70 cm length of the superconducting cable); accelerator magnets normally work at 80%, or less, of the short-sample value. The results of the 1–m LHC models also made it clear that the cable’s mechanical and electrical characteristics and the field quality of the magnet (both during ramp and at the flat top) were not far from the quality required for the LHC.
A final step would be to combine the two branches of the development work and put together magnets of the twin design with a 10 m cold mass in a 1.8 K cryostat to demonstrate that full-size, LHC dipoles of the final design were feasible. However, the strict deadline imposed by the then director-general, Rubbia, dictated that the LHC should have the same time-scale as the SSC and be ready at the end of 1999. This meant that CERN was forced to launch the programme for the first full-size LHC prototypes in 1988, i.e. well before the end of the previous step, the construction in parallel of 1 m LHC MTA models and the 10-m long TAP.
At this point, CERN was just finishing construction of LEP and beginning work on industrialization of the components for LEP2; it was a period of shortage of personnel and financial resources (not a new situation). So Brianti and collaborators devised a new strategy: for the first time CERN would seek strong participation from national institutes in member states in the accelerator R&D and construction. In 1987–1988 the president of INFN, Nicola Cabibbo, and CERN’s director-general, Schopper, agreed – with a simple exchange of letters (everything was easier in those days) – that INFN would given an exceptional contribution to the LHC R&D phase. The total value was about SwFr12 million (1990 values) to be spread over eight years.
Towards real prototypes
In 1988 and 1989, INFN and CERN ordered LHC-type superconducting cables for long magnets and in 1989 INFN ordered two 10-m long twin dipoles from Ansaldo Componenti in Italy, some nine months before CERN had the budget to order three long dipoles, one from Ansaldo and two from Noell, a German company that had been involved in the construction of HERA quadrupoles. A fourth CERN long magnet, without the twin design, was ordered from the newly formed Alstom-Jeumont consortium (even at CERN some people still doubted the effectiveness of the twin design). The effort was decisive in being able by 1993 to have the magnets qualified by individual tests and then put into a string, consisting of dipoles and quadrupoles connected in series to simulate the behaviour of a basic LHC cell.
Parallel to the INFN effort, the French CEA-Saclay institute established collaboration with CERN and took over the construction of the first two full-size superconducting quadrupoles for the LHC. While CERN provided specifications and all of the magnet components (including superconducting cable), CEA did the full design and assembly of these quadrupoles, for a value a few million Swiss francs over the eight years of R&D (CERN Courier January/February 2007 p25). This was the start of a long collaboration; the French also continued to support the project after the initial R&D, throughout industrialization and construction phases, with an in-kind contribution on quadrupoles, cryostats and cryogenics for about SwFr50 million (split between CEA and CNRS-IN2P3).
The challenge of the prototyping was hard and covered many aspects. In particular for the dipoles, CERN first had to convince industry to pay enough attention and to invest resources in the LHC; the allure of the SSC, a much larger project (6000 main dipoles of 15 m length, 2000 quadrupoles, etc), was difficult to ignore. CERN’s project was much more challenging technically, with the required accuracy of the tooling a factor of five or so higher than for the HERA magnets. There was also the usual fight in a prototyping phase: good results required building expensive tooling for one or two magnets, with insufficient budget and no certainty that the project would be approved and the tooling cost thus paid for.

A delay of one year was the price to pay for the many developments and adjustments. Meanwhile, in 1993 the project had to pass a tough review devoted to the cryo-magnet system led by Robert Aymar, who as CERN’s director-general 10 years later would collect the fruit of the review. With the review over and completion of the long magnet prototypes approaching, the credibility of the LHC project increased. In autumn 1993, the SSC came to a halt – certainly because of high and increasing cost (more than $12 billion) and the low economic cycle in the US, but also because the LHC now seemed a credible alternative to reach similar goals at a much lower cost ($2 billion in CERN accounting). Rubbia, near the end of his mandate as director-general, which was the most critical for the R&D phase, led the project without rival. In a symbolic coincidence, the demise of the SSC occurred at the same as leadership of the LHC project passed from Giorgio Brianti, who had led the project firmly from its birth through the years of uncertainty, to Lyn Evans, who was to be in charge until completion 15 years later. The end of the SSC and the green light for the LHC was marked by the delivery to CERN of the first INFN dipole magnet in December 1993, just in time to be shown to the Council. This was followed four months later by the second INFN magnet and then by the CERN magnets, as well as by the two CEA quadrupoles designed and built by the team of Jacques Perot and later Jean-Michel Rifflet (figure 4).
Returning to the first dipole, which had been delivered from INFN at the end of 1993, a crash programme was necessary to overcome an unexpected problem (a short circuit in the busbar system – a problem that in a different form would later plague the project), so as to test it by in time for a special April session of the Council in 1994. The magnet passed with flying colours, going above the operational field of 8.4 T at the first quench, beyond 9 T in two quenches, and a first quench above 9 T after a thermal cycle i.e. full memory (figure 5). Its better-than-expected performance was actually misleading, giving the idea that construction of the LHC might be easy; in fact, it took a long six years before another equally good magnet was again on the CERN test bench. However, the other 10-m long magnets performed reasonably well and with the two very good CEA quadrupoles (3.5 m long), CERN set up the first LHC magnet string, to test it thoroughly and finally receive the approval of the project in December 1994.
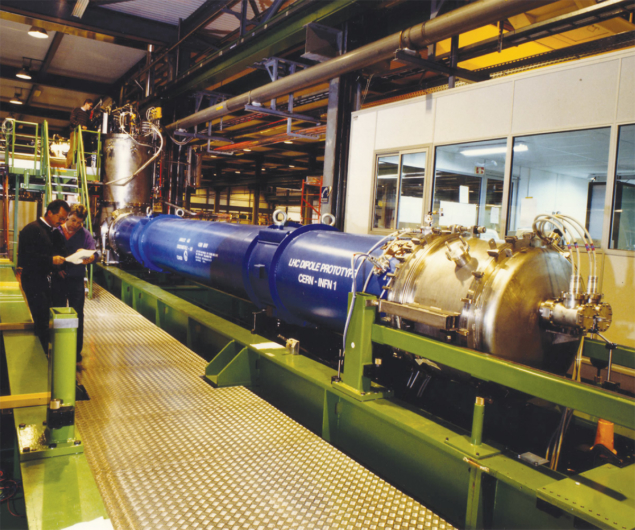
Many other formidable challenges were still to be resolved on the technical, managerial and financial sides. These included: the nonuniformity of quench results and the problem of retraining that plagued the second generation of LHC prototypes; the unresolved question of the inter-strand resistance; the change of aluminium to austenitic steel as the material for the collars, implemented by Carlo Wyss; and the lengthening of the magnets from 10 m to 15 m with the consequent curvature of the cold mass, etc.
Looking back at the period 1985–1994, when the base for the LHC was established, it is clear that a big leap forward was accomplished during those years. The vision initiated by Robert Wilson for the Tevatron was brought to a peak, pushing the limit of Nb-Ti to its extreme on a large scale. New superconducting cables, new superconducting magnet architectures and new cooling schemes were put to the test, in the constant search for economic solutions that would be applicable later to large scale production. This last point is an important heritage that the LHC leaves to the world of superconductivity: the best performing solution is not always going to be really the best. Economics and large-scale production are very important when a magnet is part of a large system and integration is critical. “The best is the enemy of the good” has been the guiding maxim of the LHC project – a lesson from the LHC for the world of superconductivity in this 100th anniversary year.