In the May 2007 issue, Nick Jelley (University of Oxford) and Alan Poon (Lawrence Berkeley National Laboratory) looked back at the achievements of the SNO experiment, which helped reveal a new world of massive neutrinos.

The end of an era came on 28 November 2006 when the Sudbury Neutrino Observatory (SNO) stopped data-taking after eight years of exciting discoveries. During this time the observatory saw evidence that neutrinos, produced in the fusion of hydrogen in the solar core, change type – or flavour – while passing through the Sun on their way to Earth. This observation explained the long-standing puzzle as to why previous experiments had seen fewer solar neutrinos than predicted and also confirmed that these elusive particles have mass.
Ray Davis’s radiochemical experiment first detected solar neutrinos in 1967, a discovery for which he shared the 2002 Nobel Prize in Physics (CERN Courier December 2002 p15). Surprisingly, he found only about a third of the number predicted from models of the Sun’s output. The Kamiokande II experiment in Japan confirmed this deficit, which became known as the solar-neutrino problem, while other detectors saw related shortfalls in the number of solar neutrinos. A possible explanation, suggested by Vladimir Gribov and Bruno Pontecorvo in 1969, was that some of the electron-neutrinos, which are produced in the Sun, “oscillated” into neutrinos that could not be detected in Davis’s detector. This oscillation mechanism requires that neutrinos have non-zero mass.
In 1985, the late Herb Chen pointed out that heavy water (D2O) has a unique advantage when it comes to detecting the neutrinos from 8B decays in the solar-fusion process, as it enables both the number of electron neutrinos and the number of all types of neutrinos to be measured. In heavy water neutrinos of all types can break a deuteron into its constituent proton and neutron (the neutral-current reaction), while only electron neutrinos can change the deuteron into two protons and release an electron (the charged-current reaction). A comparison of the flux of electron neutrinos with that of all flavours can then reveal whether flavour transformation is the cause of the solar-neutrino deficit. This is the principle behind the SNO experiment.
International collaboration
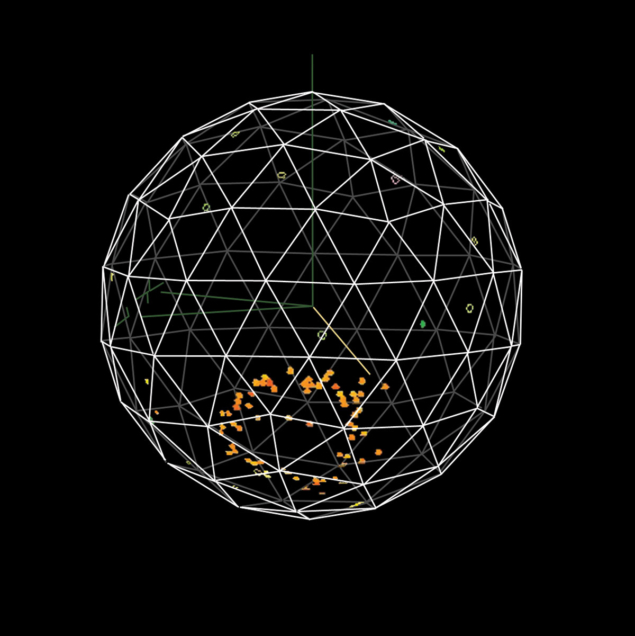
Scientists from Canada, the US and the UK designed SNO to attain a detection rate of about 10 solar neutrinos a day using 1000 tonnes of heavy water. Neutrino interactions were detected by 9456 photomultiplier tubes surrounding the heavy water, which was contained in a 12 m diameter acrylic sphere. This sphere was surrounded by 7000 tonnes of ultra-pure water to shield against radioactivity. Figure 1 shows the layout of the SNO detector, which is located about 2 km underground in Inco’s Creighton nickel mine near Sudbury, Canada, so as to all but eliminate cosmic rays from reaching the detector. Figure 2 shows what the detector “sees”: the photo-multiplier tubes that were hit following the creation of an electron by an electron neutrino.
It was crucial to the success of this experiment to make the components of SNO very clean and, in particular, to reduce the radio-activity within the heavy water to exceedingly low levels. To achieve this aim the team constructed the detector in a Class-2000 cleanroom and entry to SNO was via a shower and changing rooms to reduce the chance of any dust contamination from the mine. The fraction of natural thorium in the D2O had to be less than a few parts in 1015, roughly equivalent to a small teaspoonful of rock dust added to the 1000 tonnes of heavy water. Such purity was necessary to reduce the break-up of deuterons by gamma rays from natural uranium and thorium radioactivity to a small fraction of the rate from the solar neutrinos. This required complex water purification and assay systems to reduce and measure the radioactivity. Great care in handling the heavy water was also needed as it is on loan from Atomic Energy of Canada Ltd (AECL) and is worth about C$300 million.
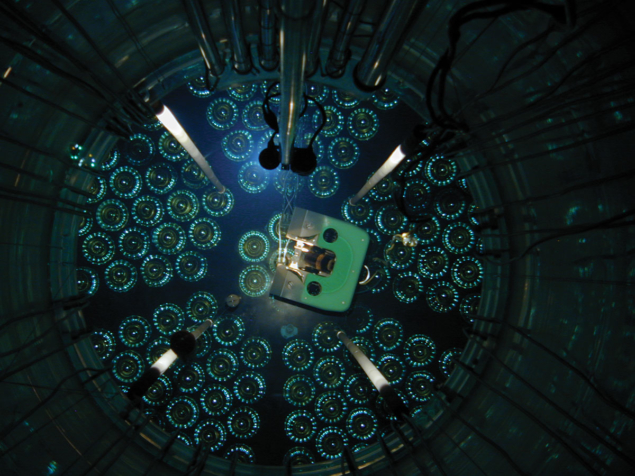
SNO’s results from the first phase of data-taking with unadulterated D2O were published in 2001 and 2002, and provided strong evidence that electron neutrinos do transform into other types of neutrino (CERN Courier June 2002 p5). The second phase of SNO involved adding 2 tonnes of table salt (NaCl) to the D2O to enhance the detection efficiency for neutrons. This large “pinch of salt” enabled SNO to make the most direct and precise measurement of the total number of solar neutrinos, which is in excellent agreement with solar-model calculations (CERN Courier November 2003 p5). The results to date reject the null hypothesis of no neutrino flavour change by more than 7 σ.
Together with other solar-neutrino measurements, the SNO results are best described by neutrino oscillation enhanced by neutrinos interacting with matter as they pass through the Sun – a resonant effect that Stanislav Mikheyev, Alexei Smirnov and Lincoln Wolfenstein predicted in 1985. To a good approximation, the electron-neutrino flavour eigenstate is a linear combination of two mass eigenstates with masses m1 and m2. The mixing angle between these two mass eigenstates, which the ratio (measured by SNO) of the electron-neutrino flux to the total neutrino flux constrains, is found to be large (around 34°) but is excluded from maximal mixing (45°) by more than 5 σ. The matter enhancement enables the ordering (hierarchy) of the two mass eigenstates to be defined, with m2 > m1 and a difference of around 0.01 eV/c2. The KamLAND experiment, which uses 1000 tonnes of liquid scintillator to detect anti-neutrinos from Japan’s nuclear reactors, confirmed in 2003 that neutrino mixing occurs and is large, as seen for solar neutrinos.
After the removal of salt from the heavy water, the third and final phase of SNO used an array of proportional counters in the heavy water to improve further the neutrino detection. Researchers filled 36 counters with 3He and four with 4He gas. Figure 3 shows part of this array during its deployment with a remotely operated submarine. The additional information from this phase will enable the SNO collaboration to determine better the oscillation parameters that describe the neutrino mixing. Data analysis is still in progress.
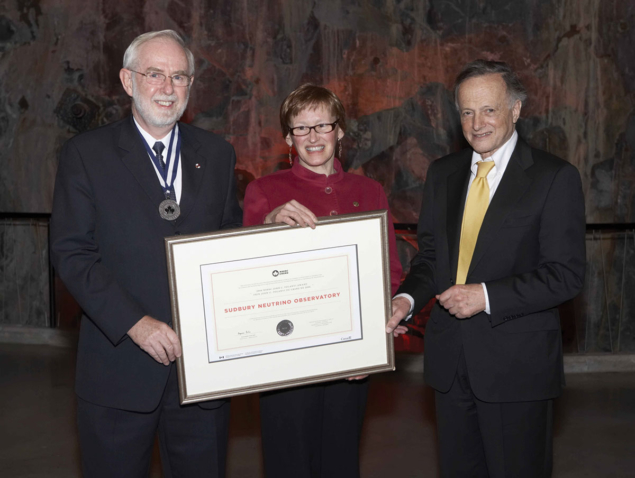
SNO’s scientific achievements were marked at the end of data-taking when the collaboration received the inaugural John C Polanyi Award (figure 4) of the Canadian funding agency, the Natural Sciences and Engineering Research Council (NSERC). The completion of SNO does not mark the end of experiments in Sudbury, however, as SNOLAB, a new international underground laboratory, is nearly complete, with expanded space to accommodate four or more experiments (see Canada looks to future of subatomic physics). SNOLAB has received a number of letters of interest from experiments on dark matter, double beta decay, supernovae and solar neutrinos. In addition, a new collaboration is planning to put 1000 tonnes of scintillator in the SNO acrylic vessel once the heavy water is returned to the AECL by the end of 2007. This experiment, called SNO+, aims to study lower-energy solar neutrinos from the “pep” reaction in the proton–proton chain, and to study the double beta decay of 150Nd by the addition of a metallo-organic compound.
As a historical anecdote, SNO was not the first heavy-water solar-neutrino experiment. In 1965, Tom Jenkins, along with other members of Fred Reines’ neutrino group, at what was then the Case Institute of Technology, began the construction of a 2 tonne heavy-water Cherenkov detector, complete with 55 photomultiplier tubes, in the Morton salt mine in Ohio. Unlike Chen’s proposal, Jenkins had only considered the detection of electron neutrinos through the charged-current reaction as other flavours were not expected, and the neutral-current reaction had not yet been discovered. This experiment finished in 1968 after Davis had obtained a much lower 8B solar-neutrino flux than had been predicted.
- This article was adapted from text in CERN Courier vol. 47, May 2007, pp26–28