What do you get when you take 1 Gt of water, cool it to -40 °C and add 4800 phototubes? The answer is IceCube, a 1 km3 neutrino telescope now being deployed at the South Pole.
The IceCube observatory is being built to detect extraterrestrial neutrinos with energies above 100 GeV. Neutrinos are attractive for high-energy astronomy because they are not absorbed in dense sources like other probe particles, and they travel in straight lines from their source. Protons or nuclear cosmic rays are also bent by interstellar magnetic fields, and while photons fly straight, at energies above 10 TeV, their interactions (by e+e– pair creation) with interstellar background photons limit their range.
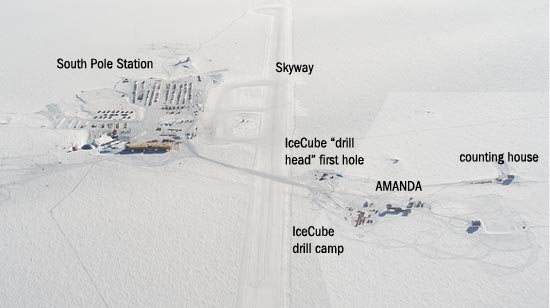
The interaction cross-sections of neutrinos are tiny, so a huge detector is required. Calculations of neutrino production in many different types of sources show that a 1 km3 (1 Gt) detector is required to observe astrophysical signals. IceCube will observe neutrinos that interact in Antarctic ice at the South Pole, producing muons, electrons or tau particles. These leptons interact with the ice (and the tau also decays), producing additional charged particles. High-energy (peta-electron-volts) muons travel many kilometres in the ice, and IceCube will observe muons that traverse the detector. The charged particles emit optical Cherenkov radiation, which can travel hundreds of metres before being detected by IceCube’s digital optical modules (DOMs). The type of neutrino, its direction and its energy can then be reconstructed by measuring the intensity and arrival time of the light at many DOMs.
Work at the South Pole
The South Pole might seem like an unusual place to build a huge detector, but the Antarctic ice is very clear and very stable. Deep below the surface, the light-absorption length can exceed 250 m. Compared with seawater, which is another active medium, the ice has much lower levels of background radiation and a longer attenuation length, but more light is scattered.
Using the US South Pole station as a base for operations, deployment of the IceCube detector in the ice began on 15 January, when the first hole was started. A jet of water heated to 90 °C was used to melt the hole. The drill pumped 750 l/min of water from a 5 MW heater to reach a drilling speed of slightly over 1 m/min. Drilling this first hole, 2450 m deep and 60 cm in diameter, took about 52 h. Once the drilling was complete, a string of 60 DOMs was lowered into the hole, which took another 20 h. The DOMs are attached to the string every 17 m, between depths of 1450 and 2450 m. The water that remained in the hole took about two weeks to freeze.
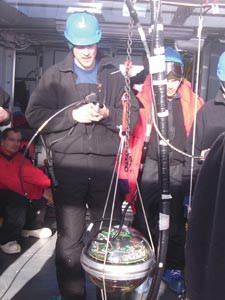
The South Pole is very different from an accelerator laboratory, and logistics is a key issue for IceCube. Environmental conditions are rough, and manpower and working time are limited, so everything must be carefully engineered and tested before being shipped to the Pole. Everything must be flown in from the Antarctic coast on LC-130 turboprop aeroplanes equipped with skis. The drilling rig alone filled 30 flights, about an eighth of the annual capacity; fuel for the drill required another 25 flights. Many of the components were transported in pieces to the Pole. The reassembly time limited this inaugural drilling campaign to about 10 days.
The image below shows an early result of this hard work, a cosmic-ray muon in IceCube, in coincidence with an air shower observed by eight surface tanks that form part of the IceTop array above IceCube. These data were taken less than two weeks after deployment, showing that everything works “right out of the box”. At the time, many of the DOMs were not yet turned on. More recent tests have verified that all 60 DOMs are working.
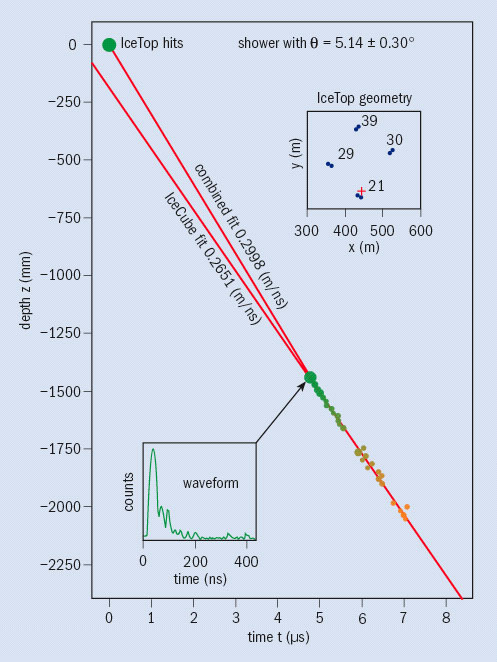
This success owed much to the Antarctic Muon and Neutrino Detector Array (AMANDA), which preceded IceCube and had more than 650 modules. The AMANDA optical modules contained only a photomultiplier tube (PMT) and analogue signals were transmitted to the surface on the power cable; later versions used fibre-optic cables to transmit analogue signals. These schemes worked in AMANDA, which observed several thousand atmospheric neutrinos, but the approach required manpower-intensive calibrations and could not be scaled up for the much larger IceCube. The solution was “String 18”, a string of DOMs that, in addition to the AMANDA fibre readout, included electronics for locally digitizing the signals, and sending digital signals to the surface. The digital readout worked, and the DOM approach was adopted by IceCube. This advance was a key to reaching the 1 km3 scale.
Each DOM functions independently. Data collection starts when a photon is detected. The PMT output is collected with a custom waveform-digitizer chip, which samples the signal 128 times at 300 megasamples per second. Three parallel 10 bit digitizers combine to provide a dynamic range of 16 bits. Late-arriving light is recorded with a 40 MHz, 10 bit analogue-to-digital converter, which stores 256 samples (6.4 μs). These waveforms enable IceCube to reconstruct the arrival time of most detected photons. A large field-programmable gate array with an embedded processor controls the system, compresses the data and forms it into packets.
The entire DOM uses only 5 W of power. Adjacent DOMs communicate via local-coincidence cables, allowing for possible coincidence triggers. Data are transmitted to the surface over the DOM power cables, and at the surface trigger conditions for the strings and array-wide are applied. The data are stored locally and selected samples transmitted to the Northern Hemisphere via satellite link.
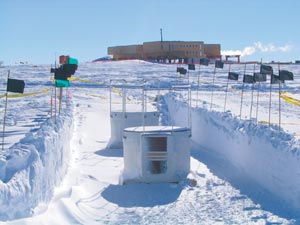
A surface cosmic-ray air-shower detector array, IceTop, forms part of IceCube. IceTop will eventually consist of 160 ice-filled tanks 2 m in diameter, distributed over 1 km2. The tanks are similar to the water tanks used in other air-shower arrays – such as at Haverah Park in the UK, the Milagro Gamma Ray Observatory in the US and the Pierre Auger Observatory in Argentina. Each tank contains two DOMs frozen in the ice. DOMs in each pair of tanks are connected via local coincidence signals, providing a simple local trigger. Part of IceTop was the first piece of IceCube to be deployed, with eight tanks installed in December 2004.
IceTop will serve several functions: tagging IceCube events that are accompanied by cosmic-ray air showers; studying the cosmic-ray composition up to around 1018 eV (correlating IceTop showers with IceCube muons); and as a calibration source to tag directionally the cosmic-ray muons that reach IceCube.
One big problem in large arrays is measuring the relative timing between separated detector elements. IceCube solved this with “RapCal”, a timing calibration whereby signals are sent down the cables from the surface then retransmitted to the surface. Accuracy is maintained by using identical electronics at the two ends of the cables. In IceCube, laboratory measurements and early data show that the local DOM clocks are kept calibrated to about 2 ns.
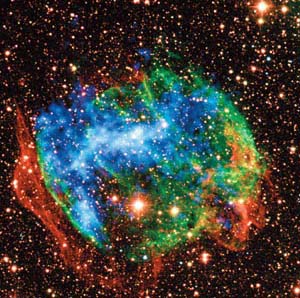
The environment at the South Pole motivated extensive reliability engineering and pre-deployment testing. The extended temperature range – down to -55 °C – was a challenge for the selection of parts and for design verification. Indeed, IceTop may reach temperatures below -55 °C, beyond the design range of any electronic components. Reliability estimates were also challenging. Conventional models predict that the failure rate halves for each 10 °C drop in temperature; according to these models, IceCube will last forever.
The physics of IceCube
IceCube will study many physics topics, but the major objective is high-energy neutrino astronomy. Any source that accelerates protons or heavier ions (cosmic rays) also produces neutrinos. The accelerated particles will collide with other nuclei, producing hadronic showers. Pions and kaons in the shower will decay, emitting neutrinos. Cosmic rays have been observed with energies up to 3 x 1020 eV and the neutrino spectrum should extend to a few per cent of this energy. The neutrino flux is model-dependent, but most calculations predict that a 1 km3 detector should see at least a handful of events each year.
There are several likely astrophysical sources of neutrinos. These include active galactic nuclei (AGNs), gamma-ray bursters (GRBs) and supernova remnants. AGNs are galaxies with massive black holes at their centres, which can power a jet of relativistic particles. Calculations based on the observed flux of photons at energies of tera-electron-volts show that IceCube should observe neutrinos from AGNs. GRBs are mysterious objects that produce bursts of high-energy gamma rays. They are associated with objects in galaxies, including hypernovae (very large supernovae) and colliding neutron stars. Some calculations suggest that IceCube should see a handful of neutrinos from a single GRB, which would be a striking result. Supernova remnants such as the Crab Nebula are the likely source of most cosmic rays of moderate energy in our galaxy. If this is correct, they must also produce neutrinos.
Neutrinos also probe cosmic rays more directly. Ultra-high-energy (above 5 x 1019 eV) protons interact with relic microwave photons from the big bang. These protons are excited into Δ resonances, which decay to lower-energy nucleons and pions. Subsequent pion and neutron decays then produce neutrinos. The proton energy-loss limits the range of very energetic protons; this is known as the Greisen-Kuzmin-Zatsepin cutoff. Photo-dissociation plays a similar role for heavier ions, limiting their range and producing neutrinos. By measuring the ultra-high-energy neutrino spectrum, IceCube will probe the cosmic-ray composition and possible evolution (with redshift) of energetic cosmic-ray sources.
Besides being two orders of magnitude larger, IceCube has several advantages over experiments such as AMANDA and the array in Lake Baikal. IceCube is optimized for higher-energy neutrinos (especially above 1 TeV), where the atmospheric neutrino background is lower. The high detector granularity will allow IceCube to study electron-neutrinos and tau-neutrinos as well as muon-neutrinos. The electron-neutrinos produce blob-like electromagnetic showers, which contrast strongly with long muon tracks; the latter can extend for many kilometres. Above 1015 eV, tau-neutrinos are identifiable through their distinctive “double-bang” signature – an initial shower from the tau-neutrino interaction and the single track of a tau particle, which eventually decays, producing a second shower.
IceCube will study many other physics topics. Over a decade, it will observe about 1 million atmospheric neutrinos, enough to search for deviations from the standard three-flavour scenario for neutrino oscillations. The IceCube collaboration will also look for violations of the Lorentz and equivalence principles, and will search for neutrinos produced by the annihilation of weakly interacting massive particles that have been gravitationally captured by the Earth or the Sun. Because of the very low dark-noise rates (about 800 Hz per DOM), IceCube can detect bursts of low-energy neutrinos from collapsing supernovae. The detector will also contribute to glaciology, studying the dust layers that record the Earth’s weather over the past 200,000 years.
• The IceCube collaboration consists of more than 150 scientists, engineers and computer scientists from the US, Belgium, Germany, Japan, the Netherlands, New Zealand, Sweden and the UK. IceCube is funded by a $242 million Major Research Equipment Grant from the US National Science Foundation, plus approximately $30 million from European funding agencies.
Further reading
J Ahrens et al. 2004 Astropart. Phys. 20 507.
E Andres et al. 2001 Nature 410 6827.
See also www.icecube.wisc.edu.