Exploring the Higgs boson’s couplings to other particles and the shape of its potential could be the key to physics beyond the Standard Model. But only a future collider can fully open such vistas, explains Laura Reina.
Many of the most arbitrary aspects of the Standard Model of particle physics (SM) are intimately connected to the scalar sector of the theory. The SM comprises just one scalar particle, the Higgs boson, and assumes a specific scalar potential (the famous “Mexican hat”) to define the dynamics of electroweak (EW) interactions. But the fact that the Higgs boson acquires a non-zero vacuum expectation value that defines the mass scale of EW interactions (around 100–200 GeV) is assumed, not explained, by the SM. Indeed, why the Higgs-boson mass is constrained to be at the EW scale, while quantum corrections should push it to much higher values (the so-called naturalness problem, see Naturalness after the Higgs), is not justified by any symmetry of the SM. At the same time, the SM assumes that fermion masses are generated via arbitrary Yukawa-type interactions with the scalar field but it does not explain the hierarchy of couplings or masses that we observe, nor the specific flavour structure that arises from the presence of just one scalar field.
Future colliders are vital to push the precision Higgs programme to the next level
The scalar sector of the SM may therefore be seen as a messenger of a more fundamental theory that replaces the SM at energies beyond the EW scale and turns apparent arbitrariness into logical consequences. After all, the mechanism of EW symmetry breaking as realised in the SM via the Brout–Englert–Higgs (BEH) field is just the simplest possible way to generate massive EW gauge bosons and fermions while preserving gauge symmetry. The scalar potential could be more complicated, for example involving multiple scalar fields, as is common in many beyond-the-SM (BSM) theories. This would result in a richer pattern of stable and metastable minima and influence the nature of the EW phase transition. A first-order phase transition, together with extra sources of CP violation beyond what is implied by the SM, could explain the origin of the matter–antimatter asymmetry of the universe via EW baryogenesis (see Electroweak baryogenesis). Understanding the origin of the EW scale is thus key to connecting very different realms of particle physics and cosmology, and the question we face while we look into the future of collider physics.
Game changer
The discovery of the Higgs boson during Run 1 of the LHC has been a game changer in the exploration of new physics beyond the EW scale. The measurement of the Higgs-boson mass has added the last missing input parameter to precision global fits of the SM, which now provide a very powerful tool to constrain BSM scenarios. Thanks to an unprecedented level of precision reached in both theory and experiment, the measurement of Higgs-boson couplings to EW gauge bosons (W, Z) and to the first two generations of quarks and leptons (t, b, τ, µ) from Run 2 data has already constrained their deviations from SM expectations to within 5–20%, with the best accuracy reached for the couplings to the gauge bosons. Based on these results, the High-Luminosity LHC (HL-LHC) is projected to constrain the effects of new physics on Higgs-boson couplings to EW gauge bosons to 1–2%, and to heavy quarks and fermions to 3–5%. If no anomalies are found, this level of accuracy will push the lower bound on the scale of new physics into the TeV ballpark. Vice versa, the detection of possible anomalies may point to the presence of new physics at the TeV scale, possibly just around the corner.

On the other hand, testing the SM scalar potential will still be challenging even during the HL-LHC era. The shape of the BEH potential can be tested by measuring the Higgs-boson self-interactions corresponding to its cubic and quartic terms. In the SM, these interactions are strictly proportional to the Higgs-boson mass via the vacuum expectation value of the BEH field. Deviations from the SM are searched for via Higgs pair production and radiative corrections to single-Higgs measurements. Although the LHC and HL-LHC promise to provide evidence for di-Higgs production, the extraction of the Higgs self-coupling from such measurements will be statistically limited.
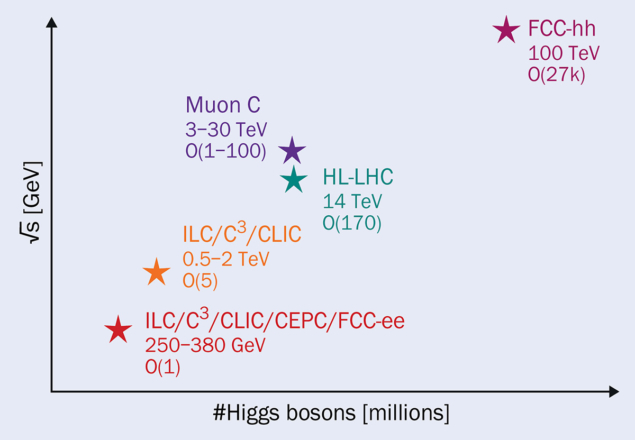
Future colliders are vital to push the precision Higgs programme to the next level. While the type and concept of the next collider is yet to be decided, all proposed facilities would deliver a huge number of Higgs bosons over their lifetime, operating at different and well targeted centre-of-mass energies (see “At a glance” figure). They can complement one another and, staggered over a period of the next few decades, provide the missing elements of the EW puzzle.
Among future lepton colliders under study, circular e+e– colliders (CEPC, FCC-ee) are expected to operate at lower energies between 90–350 GeV with very high luminosities, while linear e+e– colliders (ILC, C3, CLIC) offer both low- and high-energy phases generally with slightly lower luminosities. Combined with data from the HL-LHC, these “Higgs factories” would enable the SM, including most Higgs couplings, to be stress-tested below the per-cent level and in cases at or below the per-mille level. In particular, FCC-ee operating at the s-channel Higgs resonance (125 GeV) has the capability to provide bounds on couplings as small as the electron Yukawa coupling, while linear e+e– colliders operating at 550–600 GeV and above could substantially improve on the top-quark Yukawa coupling with respect to the HL-LHC. A possible muon collider, operated either as a Higgs factory at 125 GeV or as a high-energy discovery machine at 3–10 TeV, is estimated to reach similar precisions on Higgs couplings to other particles as e+e– machines.

Finally, high-energy lepton colliders (ILC 1000, CLIC 3000 and a 3–30 TeV muon collider) and very high-energy hadron colliders (FCC-hh at 100 TeV) would reach enough statistics and energy to measure the Higgs self-coupling and investigate the nature of the BEH potential, either via di-Higgs or single-Higgs production (see “Self-coupling” figure). With an aggressive Higgs physics programme they may also reach enough sensitivity to probe the cubic and quartic terms in the BEH potential separately.
Almost half a century after it was predicted, the LHC delivered the Higgs boson in spectacular style on 4 July 2012. Over the next 15–20 years, the machine and its luminosity upgrade will continue to enable ATLAS and CMS to make great strides in understanding the Higgs boson’s properties. But to fully exploit the discovery of the Higgs boson and explore its mysterious relation to new physics beyond the EW scale, we will need a successor collider.