In 1900 nobody could have even dreamed of the way in which science would evolve over the 100 years that followed. Theorist and science writer Frank Close reviews the past century and looks forward to the next.
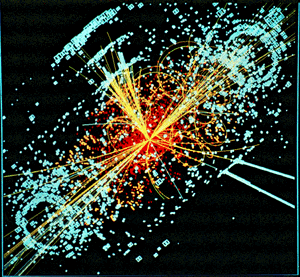
If the structure of DNA and the nature of life qualify as the most profound discoveries of the 20th century, what will be those of the next? Such are the questions beloved by pundits as the calendar turns in this special year. We know that these questions are unanswerable. However, to appreciate quite how unpredictable the future is, it may be worth imagining that we were being asked such a question 100 years ago.
In the late 19th century some scientists believed that the basic principles of physics had been discovered and only the details remained to be worked through. An outstanding problem – the apparently perverse behaviour of the spectrum of radiation emitted by hot bodies – was solved 100 years ago with the invention of quantum theory. Our view of the world was utterly changed. Can we draw any parallels with the present? Perhaps the writer of a similar article 100 years from now will give the answer.
As the 19th century drew to its end, three great discoveries defined the 20th century and illuminated the nascent science that we now call particle physics. In less than three years, between late 1895 and 1897, Röntgen discovered X-rays, Becquerel found radioactivity and Thomson isolated the electron. For me, the discovery of X rays and the electron typify the one hundred year leap from then to where we are today.
Ask a member of the general public about X-rays and they will think of shadows of broken bones: ask a scientist, and they will point to X-ray crystallography. As Röntgen prepared to receive the first Nobel prize in physics in 1901, no-one foresaw Bragg’s work in X-ray crystallography, let alone that, half a century later, Crick and Watson would use this tool to resolve the structure of DNA. What many would regard as the most profound discovery in biology is, by many readers of CERN Courier, recognized as applied physics.
Genetics in the 21st century is likely to be as revolutionary as electronics has been in the 20th century. It is electronics, and all else that has flowed from the discovery of the electron, that touches most people in our field today.
Nature’s fundamental pieces
J J Thomson marched into the Royal Institution on the 30 April 1897 and announced his discovery of the electron, a fundamental constituent of all atomic elements. After Thomson duly won a Nobel prize (1906), for showing that the electron is a particle, his son, G P Thomson subsequently won the prize in 1937, for showing that the electron is a wave. That, however, is another story.
Jump forward in time to the late 1960s, and beams of electrons, accelerated over a distance of 3 km, were fired into targets of protons and neutrons at SLAC. These experiments showed that the cosmic onion does not end with the atomic nucleus. The ultimate nuclear constituents (for the 20th century at least) are the quarks.
The century had begun with the belief that atomic elements were nature’s fundamental pieces. It ended with the discovery of electrons and quarks. The electron is but one member of a family of six, known as leptons; there are six varieties of quark as well. No-one at the end of the 20th century knows for certain why six of one and half a dozen of the other is nature’s scheme, but the answer will probably be known by the end of the 21st century.
In 1897 J J Thomson, alone in his Cambridge laboratory, discovered the electron by means of a small glass tube, which was less than 27 cm long. By 1997 electrons were speeding around CERN’s LEP ring (a journey of 27 km) to meet their nemesis, positrons, which were unknown to Thomson but, mysteriously, known to mathematics before their discovery by humans.

Antimatter
It was with the discovery of the positron, and the anti-world, that the electron revealed the deep power of mathematics. In 1928, Paul Dirac took the two great theories of the 20th century – relativity and quantum mechanics – and applied them to the electron. The mathematics simply would not balance.
The greatest implication of Dirac’s equation (as it will be known for all time) was that it opened a window to an entirely new world. His equation had two solutions, one being the familiar negatively charged electron, while the other implied the existence of a bizarre mirror-image version, identical in all respects except that the sign of its electrical charge is positive rather than negative. This anti-electron, more succinctly known as the positron (positive electron), is an example of antimatter.
Dirac’s prediction of the positron seemed to many at the time to be science fiction. Up to that point the only particles known or predicted, existed as constituents of the matter around us, namely electrons in the periphery of atoms and protons and neutrons, which comprise the atomic nucleus. The positron, which had emerged from his equations like a rabbit from a magician’s cloak, had no place at all. However, the questions ended in 1932 when the positron was found in cosmic radiation, with a positive charge and an identical mass to its electron sibling.
Dirac’s theory, that for every particle there exists an antiparticle counterpart, is now recognized to be an essential truth: a glimpse of a profound symmetry in the fundamental tapestry of the universe. And here we have another of the great puzzles that are with us at the turn of the century. If, as experiment suggests, the Big Bang created particles of matter and antimatter in equal amounts, and they annihilate upon meeting, how is it that there is any material universe remaining? Where has all the antimatter gone? Crick and Watson revealed the nature of life as we now know it, but how did the universe manage to survive long enough, made of matter, to have provided the necessary circumstances for life to emerge?
While the annihilation of matter and antimatter is a puzzle for understanding our existence, it is, nonetheless, the annihilation of the simplest pieces, electrons and positrons, that has been the key to LEP. Accelerated around the 27 km ring, the collisions of positrons electrons and their mutual annihilations produce, in a small volume, for a brief moment, energies that are far greater than are found in any star and akin to those prevalent in the universe when it was less than a billionth of a second old. Particles of matter and antimatter pour out from these “mini bangs”, replaying the basic processes that occurred at the Big Bang. To capture the fleeing particles, which are travelling close to the speed of light, huge detectors are required.
Recreating the Big Bang
It is when you stand alongside one of these behemoths and compare it with the little tube that Thomson used, that you see 100 years of science and technology in metaphor. It was relatively easy for Thomson to isolate the electron because the universe had already done much of the preparatory work. Over the previous 10 billion years, electrons had been created, trapped in atomic elements and stored there in the solid matter of the new-born Earth until we arrived. They were ubiquitous in 1897 Cambridge. A small tube and genius, then asset-stripped the atoms aided by relatively primitive tools (which used electric and magnetic forces to move the electrons around) to reveal their existence and their properties.
Today, by contrast, we are looking at exotic forms of matter: heavy quarks, supersymmetric particles and the Higgs boson, all of which, theorists believe, existed briefly in the afterglow of Creation but now are no longer here. To find them, we have to restore the conditions of the new-born universe.
There are no mass produced test tubes that can make an experiment of such magnitude. There is no customized “Big Bang apparatus” for sale in the scientific catalogues so that we can experience the first moments of the universe in our living rooms. This is not mere hype. To journey to the start of time you have to build all the pieces for yourself, by transforming the earth, rocks and gases of our planet into tools that extend our senses. That is how it has been at LEP, and how it will be for CERN’s LHC.
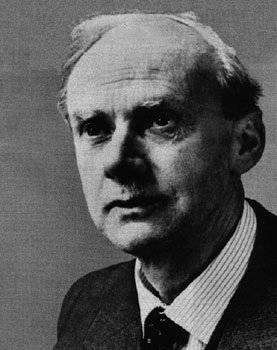
Sand provides the raw material for the nervous system of computer chips, which will orchestrate the enterprise. Hydrogen gas, from which protons can be stripped, will supply the beams for the LHC. Ores dug from the ground, melted, transformed and turned into magnets will be capable of guiding beams of protons at 99.9999% of the speed of light. A myriad of other tools, which are the result of centuries of invention, are being assembled. When all is done, these tools of the millennium will reveal the universe, not as it is now, but as it was at Creation. This is a far cry from Thomson’s day.
The results will be gathered, not by a single person as in Thomson’s case, but by a team of thousands, working on several continents, communicating via the internet, which is powered by electrons. Not only science and technology, but even the sociology of research has evolved over these past one hundred years.
Our story began with the first Nobel prizes. Becquerel’s discovery of radioactivity (1903) is where I shall complete the tale. The agents of the weak force, the cookers of the elements, were discovered at CERN in the 1980s. At LEP in the 1990s, millions of examples of these Z and W particles have been made and measured to astonishing precision. As the mathematics of Dirac revealed the existence of the positron, so has ‘t Hooft and Veltman’s theory of the weak force enabled quantitative descriptions of the measurements at LEP. LEP had insufficient energy to materialize a top quark. Nonetheless, courtesy of ‘t Hooft-Veltman mathematics, its properties can be deduced in advance of its triumphant discovery at Fermilab. Now, on the threshold of the 21st century, we are in an analogous situation with the Higgs boson. The precision of LEP and the mathematics give us foresight of another research prize.
So the theory of ‘t Hooft and Veltman, earning the final physics Nobel prize of the 20th century, may be giving us a glimpse of one of the first great breakthroughs of the 21st century. Will the discovery of the Higgs boson, and its associated phenomena, turn out precisely as the theorists expect? Or will there be some unexpected twists: the first hints of profound truths that are at present beyond our ken? Theorists throughout history have created beautiful descriptions of the universe, often with astonishing implications. Ultimately it is experiment that decides by distinguishing fact from fancy.
What will CERN Courier be celebrating in its issue 100 years hence? Röntgen, Becquerel and Thomson could not have imagined DNA, Zs and Ws, electroweak theory, the World Wide Web, nor LEP and LHC (machines that take us to the start of time). If there is any message from this that we can be sure is a guide for the coming century, it is this: prepare for surprises.