In the May 1966 issue of CERN Courier, A J Herz (Nuclear Physics Division) and W O Lock (Personnel Division) described the development of nuclear-emulsion detectors, highlighting a CERN experiment that determined the magnetic moment of the Λ0 baryon. By then, as the authors described, the heyday of emulsions was over, and the field had moved on to fewer, more specialised and complex experiments.
The possibility of detecting individual charged particles by means of a photographic emulsion was first investigated as long ago as 1910 by Kinoshita, working at Manchester University. He was continuing some earlier work by Lord Rutherford in the same field. Kinoshita showed that a single α particle was capable of rendering a silver-halide grain developable. A year later, Reinganum showed that the passage of an α particle at glancing incidence to a photographic emulsion produced, when the emulsion was developed, a row of silver grains outlining the trajectory of the particle.
This early work was carried out with the type of emulsion used for conventional photography, which had a thickness of only a few microns. It was not until around 1930 that thick-layered emulsions (about 50 microns) were produced, first in research laboratories and later (1935–1937) on a commercial scale by Ilford Ltd in England. These emulsions were exposed for some months at mountain altitudes, for example by Blau and Wambacher, and on subsequent examination ‘stars’ were found which were ascribed to the disintegration of nuclei in the emulsion caused by cosmic rays.
By 1939, the technique was recognized as a useful tool for the investigation of nuclear and cosmic-ray phenomena, but it was considered to be only a qualitative method and of limited application. The systematic investigations of Powell from 1940 onwards showed, however, that the method was capable of giving accurate quantitative results. For example, Chadwick, May, Pickavance and Powell studied in great detail the scattering in various gases of particles accelerated in a cyclotron. At the end of the war, Ilford produced a concentrated ‘nuclear-research’ emulsion containing eight times the normal amount of silver bromide per unit volume. It was in emulsions of this type exposed to cosmic rays at mountain altitudes that the pion was discovered by Powell and his colleagues in 1947.
Finally, in 1947–48, first Kodak Ltd and then Ilford produced an emulsion capable of recording the tracks of particles moving with velocities such that they suffer the minimum possible energy loss (causing minimum ionization) in passing through the emulsion. Such emulsions are commonly known as ‘electron-sensitive emulsions’; they can be purchased, if required, with thicknesses as great as 2 mm on glass or 1.2 mm as stripped emulsion, and in sizes up to 40 cm × 40 cm, or greater.
Advantages and disadvantages
Nuclear emulsion possesses one most significant advantage over all other techniques. It is capable of extraordinarily high spatial resolution. Other techniques can resolve events separated by a few millimetres; using emulsion we can resolve events separated by a few microns. This has made possible the measurement of the lifetime of the π0 meson (about 10–16 s) and is the basis of our confidence that there are no other commonly occurring unstable particles with lifetimes in the range 10–11 to 10–16 s.

There are some further advantages which are particularly significant in the case of complex experiments in which nuclear emulsion is used as a detector. First of all, it is an integrating device which can be exposed or irradiated until sufficient data have been stored in it; secondly, it can be used in very confined volumes, as will be illustrated later in this article in the discussion of the experiment to determine the magnetic moment of the ΛO hyperon.
Its chief disadvantage is that it consists of a mixture of complex nuclei – silver and bromine (as silver bromide) suspended in gelatine, which is largely carbon, nitrogen and oxygen. Further, the scanning of large volumes of emulsion, of necessity in three dimensions, is frequently very tedious. Thus, with the development of other powerful detectors such as the spark chamber and the bubble chamber (with its simple target material of hydrogen or deuterium for example) which are readily adaptable to automatic methods of analysis and data handling, nuclear-emulsion work has inevitably developed into a supplementary technique, except in certain special fields.
Use of the technique in research
There are two distinct ways in which nuclear emulsions can be used. Firstly, they may be placed in the path of particles (from an accelerator or in the cosmic radiation) and the interactions which these particles produce in the emulsion can be studied in detail. The charged pions and many of the ‘strange particles’ were first discovered, and their decay modes studied, in large stacks of emulsion exposed for considerable periods of time to the cosmic radiation (see Figure 2). The relatively small size and weight of emulsions enable them to be carried to great heights by means of free balloons. In this way, many studies have been carried out of a) the interactions of very energetic particles – energies greater than 50 GeV (see Figure 1), b) the composition of the primary cosmic radiation and c) the development of the electron–photon cascade in the high atmosphere.
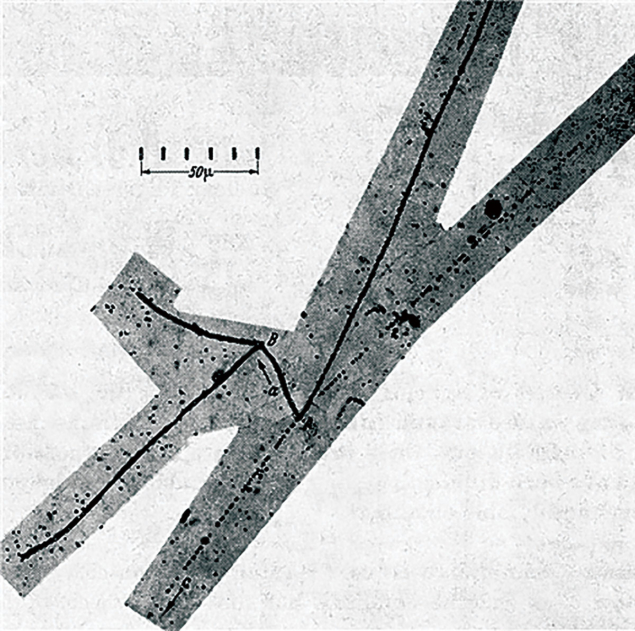
One well-known example is the work carried out by groups all over the world on emulsions which were exposed on a high-altitude balloon flight as a very large stack (60 × 45 × 30 cm) known as the ‘Schein stack’ after the American physicist who initiated the project.
The second way in which emulsion may be used is simply for particle detection, placed near to a target which is bombarded by a suitable particle beam. The target employed is often liquid hydrogen, although at cyclotron energies targets of many solids and gases have been used. In many cases the geometry of the experimental arrangement is so designed that the particles we wish to investigate stop in the emulsion, thus allowing their energy to be determined by measuring the distance they travel in the emulsion.
The ΛO magnetic moment experiment
It is thought by many that nuclear-emulsion work is somehow different from experimental research with other techniques, that it requires the application of special skills laboriously acquired during a long period of training, and that ‘emulsion workers’ spend their days looking down microscopes rather like classical botanists, making up descriptions of what they see. To judge how much truth there is in this view, we will describe one of the major experiments in which the CERN Emulsion Group has participated.
The idea that it would be possible to determine the magnetic moment of the ΛO hyperon using nuclear emulsions and a very-high-field pulsed magnet came first to V. Z. Peterson at the California Institute of Technology (CalTec), following a theoretical paper by M. Goldhaber. In 1957, Peterson proposed such an experiment, describing many of the essential features of the project finally carried out six years later. In the background of the proposal, there was the clear need to measure the magnetic moments of the hyperons. Peterson had started to develop pulsed magnets at CalTec and nuclear emulsion was suggested as the most suitable detector because of space limitations inside the magnet, coupled with a need for very high precision in the measurements on the decay products of the ΛO. The idea was carried to CERN by Ph. Rosselet, then of the University of Lausanne, who worked with Peterson on the CalTec pulsed-magnet project during 1958. After his return to Lausanne in 1959, the group there (under Professor Haenny) took up the development of pulsed-magnet coils suitable for magnetic-moment experiments, and a proposal was submitted to CERN.
It was found that an existing slow-K-meson beam could be modified to provide a flux of almost 105 π– mesons per pulse, and test exposures took place in early February 1962. Most of the information needed was obtained, and it was possible to conclude that the experiment would be feasible with a magnetic field of 150 kG instead of the 75 kG used in the test runs.

The ΛO hyperons are in the field from production to decay. They are ‘polarized’ at production such that their magnetic moments are predominantly lined up perpendicular to the plane containing the incident π– meson and the ΛO itself. The interaction between the magnetic moment and the applied field results in the rotation of this direction through an angle which is proportional to the magnetic moment and other, known, quantities such as the intensity of the field. The problem is to determine this angle Θ. It can be done because the most probable direction of emission of the pion in decay is the direction of the magnetic-moment vector. Thus if we measure the direction of emission of the pions from many ΛO decays, and transform them in each case from the laboratory system to the centre-of-mass system of the ΛO in question, we can find the average value of Θ. The precision depends on the number of ΛO decays collected, on the accuracy of measurement of direction and momentum in the emulsion, and on the errors in the determination of the value of the magnetic field and of other constants associated with the apparatus.
The main run took place, without major mishaps, in October 1963. Analysis began at the four collaborating Laboratories as soon as the processed plates had been distributed, and by Spring 1964 results were beginning to emerge. The character of the work had now changed, for the many technical tasks associated with the preparation of the experiment had been replaced by scanning, measurement and data analysis. Much comparison and discussion of data took place in meetings and by correspondence, and by June 1964 it was clear that the experiment would yield an improved estimate of the ΛO magnetic moment. A preliminary paper was read at the International Conference on High-Energy Physics held in Dubna in September. More complete results were published in Physics Letters in March 1965.
This determination of the ΛO magnetic moment is an example of an experiment using nuclear emulsions in a complex arrangement in which the application of many of the techniques of experimental physics, and some of engineering, was involved. The handling and processing of the emulsions, the subsequent scanning and the measurements, whilst crucial to the success of the project, did not constitute the major part of the work, nor, we might add, were they the most difficult.
The future
It is always risky to predict the future in print, and especially so in the present case where there is already a long record of unfulfilled pessimistic prophecy. We do not think that the emulsion technique is dead, to be soon forgotten. It is simple, easy to adapt to new requirements, and a physicist does not need a long apprenticeship in order to use it.
As with the bubble-chamber technique, the analysis of the experimental material can be done at small university laboratories, far away from the accelerator. The investment in equipment for analysis can, moreover, be relatively modest, much less than for bubble-chamber work for which access to a large computer is always needed.
Except in special applications, such as hyperfragment studies, nuclear emulsion is basically unsuitable for the investigation of very rare events and for the detection of complex processes where neutral links travel more than a few hundred microns before giving rise to a visible secondary event. Accumulated background will swamp the rare events, unless they have very striking characteristics, whilst complex processes will usually escape detection because the background obscures the relationship between vertices connected by neutral links, and because only a very small region of the emulsion can be in view at any one time.
It is clear, therefore, that future applications will be of the kind in which one or other of the strong points of the technique is of paramount importance. Such applications have always existed since the time of Kinoshita, fifty-five years ago, and we can expect that they will continue to be found in the future. For that reason, it is important not to let the emulsion technique disappear from the arsenal available to experimental physics. It may be put into storage, perhaps, but if it remains at the disposal of those who want it, it will almost certainly continue to be used from time to time.
- This article was adapted from text in CERN Courier vol. 6, May 1966, pp83–87