Chris Quigg takes a look at the enduring legacy of Fermilab’s proton–antiproton collider.
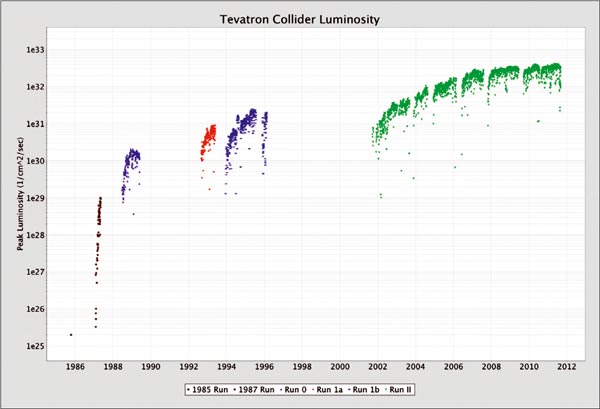
A quarter-century of experimentation is coming to a close at Fermilab’s Tevatron collider, a pioneering instrument that advanced the frontiers of accelerator science and particle physics alike, setting the stage for the LHC at CERN. The world’s first high-energy superconducting synchrotron, the Tevatron served as the model for the proton ring in the HERA collider at DESY and as a key milestone towards the development of the LHC. In its final months of operation the Tevatron’s initial luminosity for proton–antiproton collisions at 1.96 TeV averaged more than 3.5 × 1032 cm–2s–1. The integrated luminosity delivered at 1.96 TeV approached 12 fb–1, with approximately 10 fb–1 recorded by the CDF and DØ experiments. A long line of innovations and much perseverance made possible the evolution of luminosity shown in figure 1 (Holmes et al. 2011).
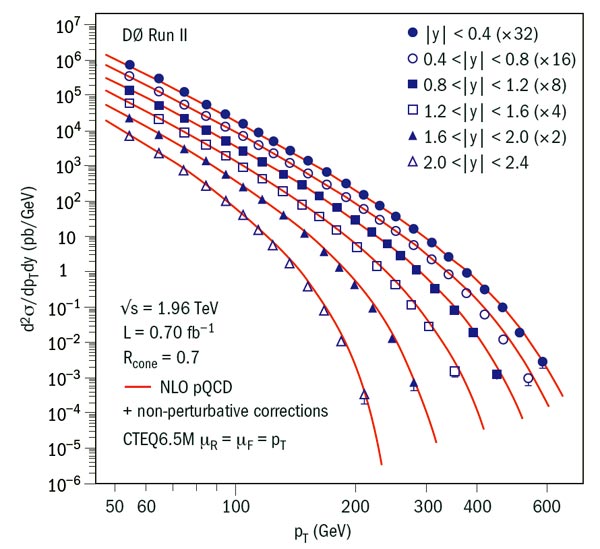
The legacy of the Tevatron experiments includes many results for which the high energy of a hadron collider was decisive. Chief among these is the discovery of the top quark, which for 15 years could be studied only at the Tevatron. Exacting measurements of the masses of the top quark and the W boson and of the frequency of Bs oscillations punctured the myth that hadron colliders are not precision instruments. Remarkable detector innovations such as the first hadron-collider silicon vertex detector and secondary vertex trigger, and multilevel triggering are now part of the standard experimental toolkit. So, too, are robust multivariate analysis techniques that enhance the sensitivity of searches in the face of challenging backgrounds. CDF and DØ exemplify one of the great strengths of particle physics: the high value of experimental collaborations whose scientific interests and capabilities expand and deepen over time – responding to new opportunities and delivering a harvest of results that were not imagined when the detectors were proposed.
Early days
The CDF logbook records the first collision event in the Tevatron at 02.32 a.m. on 13 October 1985, at an energy of 800 GeV per beam. The estimated luminosity was 2 × 1025 cm–2s–1, more than seven orders of magnitude below the machine’s performance in 2011. By the afternoon, the Tevatron complex was shut down for 18 months to construct the DØ interaction region and complete the CDF detector. CDF’s pilot run in 1987 yielded the first wave of physics papers, including measurements and searches. During 1988 and 1989 CDF accumulated 4 pb–1, now at 1.8 TeV in the centre of mass. (Two special-purpose experiments also published results from this run. Experiment 710 measured elastic scattering and the total cross-sections; Experiment 735 sought evidence of a deconfined quark–gluon plasma.) The peak luminosity delivered to CDF surpassed 1030 cm–2s–1 in collisions of six proton bunches on six antiproton bunches. Papers from these early runs are worth rereading as reminders of how little we knew, and how a tentative but growing respect for the Standard Model brought coherence to the interpretation of results. It is also interesting to see how the experimenters went about gaining confidence in their detector and their analysis techniques.
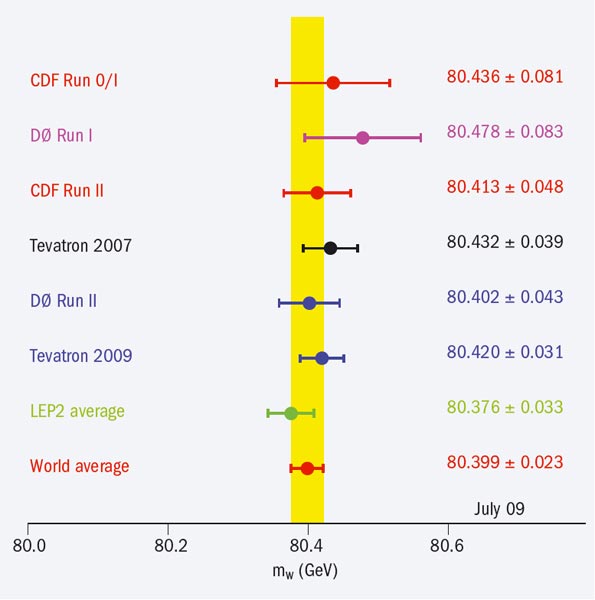
Both DØ and CDF took data at 1.8 TeV in the extended Run 1 between 1992 and 1996, recording 120 pb–1. An important enabler of increased luminosity was the move to helical orbits, which eliminated collisions outside the two interaction regions. During this period, a small test experiment called MiniMax (T864) searched for disordered chiral condensates and other novel phenomena in the far-forward region. This was a time of high excitement, not only for the drama of the top-quark search, but also for the stimulating conversation between the teams on the Tevatron experiments and those at the Z factories at CERN and SLAC, and at the HERA electron–proton collider, all of which were breaking new ground.
Fermilab then constructed the Main Injector and Recycler in a new tunnel, while the experiments undertook ambitious detector upgrades. Improvements to the cryogenic system made it possible to lower the operating temperature of the superconducting magnets and so raise the collision energy to 1.96 TeV. CDF installed a new central tracker and improved silicon vertex detector and enhanced its forward calorimetry and muon detection. DØ added a solenoid magnet, a silicon vertex detector and a scintillating-fibre tracker and also improved the detection of forward muons. Run 2 began slowly in 2001, but attention to detail and many accelerator improvements – including 36-bunch operation and electron-cooling of antiprotons in the recycler – contributed to the outstanding performance of the mature machine.
Strong and electroweak physics
The Tevatron experiments have probed the proton with a resolution of about one-third of an attometer (10–18 m), greatly expanding the kinematical range over which we can test the theory of the strong interactions. Perturbative QCD is extremely well validated in studies of hadron jets and other observables. The jet cross-section displayed in figure 2 shows the agreement between calculation and observation over eight orders of magnitude in rate (e.g. Abazov et al. 2008, Aaltonen et al. 2008 and 2009). Such measurements established the importance of gluon–gluon scattering as a mechanism for jet production and helped constrain the parton distribution functions for the gluons. Values of the strong coupling constant extracted from jet studies exhibit the running behaviour characteristic of asymptotic freedom at higher scales than accessible in other experiments. The strong coupling at the Z-boson mass has been determined with an uncertainty of about 4%.

Other jet studies have not only tested QCD but also probed for physics beyond the Standard Model. Measurements of the angular distribution of dijet production confirm the Rutherford-scattering-like expectation of QCD and place upper bounds on the size of extra spatial dimensions. They also validate, at a resolution of nearly 1/(3 TeV), a key idealization that underpins the Standard Model – the working hypothesis that quarks are pointlike and structureless. Measurements of the dijet mass spectrum that extend beyond 1.2 TeV (roughly 2/3 of the centre-of-mass energy of the proton–antiproton collisions) are likewise in accord with next-to-leading-order QCD calculations. No evidence is seen for unexpected dijet resonances.
In the final data set of 10 fb–1, each experiment should have approximately 5 million W bosons in each leptonic decay channel and perhaps 400,000 Z bosons. These large samples have made possible many important measurements. The production cross-sections agree with QCD predictions to such a degree that electroweak gauge-boson production is under study as a primary luminosity monitor for LHC experiments. Studies of Z production, with or without accompanying jets, are immensely valuable for testing simulations of Standard Model physics. The forward-backward asymmetry of the electrons or muons produced in W decay, which arises from the V–A structure of the charged weak current, provides important information about the up-quark and down-quark parton-distribution functions.
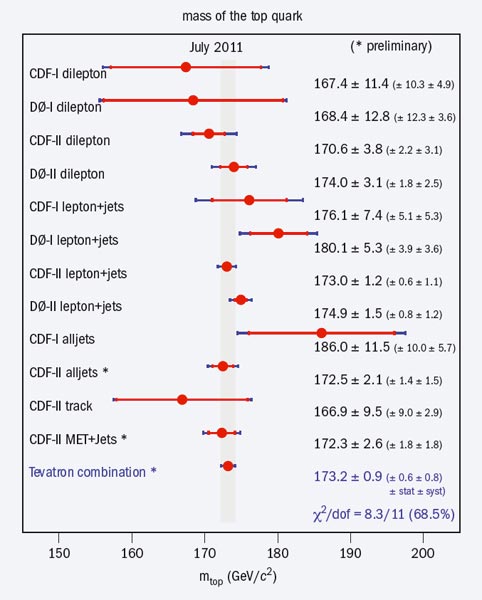
Given what we know from many sources, the masses of the W boson and top quark are key elements in the Standard Model network that constrains the properties of the Higgs boson. A stellar accomplishment of the Tevatron experiments has been the determination of the W-boson mass as 80.420 ± 0.031 GeV, better than 4 parts in 104. Figure 3 summarizes the Tevatron measurements and their impact on the current world average. The combined uncertainty at the end of Run 2 may approach 15 MeV.
The growing data samples available at the Tevatron, along with the evolution of experimental techniques, have made it possible to observe cross-sections times branching ratios well below 0.1 pb. All of the electroweak diboson pairs (Wγ, Zγ, WW, WZ and ZZ) have been detected at the rates predicted by the Standard Model. Mastery of these channels is a prerequisite to the Higgs-boson search at moderate and high masses, but they carry their own physics interest as well: the possibility of validating the Standard Model structure of the triple-gauge couplings and searching for anomalous couplings incompatible with the Standard Model. So far, the three-gauge-boson interactions are consistent with electroweak theory in every particular.
From bottom to top
CDF and DØ have exerted a broad impact on our knowledge of states containing heavy quarks. Studies of the production and decay dynamics of quarkonium states have repeatedly challenged phenomenological models, while measurements of b- and t-quark production have made possible sharp tests of QCD calculations at next-to-leading order. The Tevatron experiments account for nearly all of our knowledge of the Bc meson, with precise measurements of the mass and lifetime. The Tevatron contributes world-leading measurements of masses and lifetimes of B mesons and baryons, and has been the unique source of information on many of the B-baryons. With CDF’s recent observation of the Ξ0b, all of the spin-1/2 baryons containing one b quark have been observed at the Tevatron, except for the Σ0b. We also owe to the Tevatron our knowledge of orbitally excited B and Bs mesons, constraints on the mass and quantum numbers of X(3872), important evidence on D0–D0 mixing and high-sensitivity searches for rare decays into dimuons.
The Tevatron experiments met one of the key targets for Run 2 by determining the frequency of Bs–Bs oscillations. Following a two-sided limit published by DØ, the CDF collaboration determined the oscillation frequency as 17.77 ± 0.13 ps–1 (Abulencia et al. 2006). The oscillation signal is shown in figure 4. This beautiful measurement, in line with Standard Model expectations, constrains the manner in which new physics might show itself in B physics.
The discovery of the top quark by the Tevatron collaborations in 1995 was a landmark achievement
The discovery of the top quark by the Tevatron collaborations in 1995 was a landmark achievement (Abe et al. 1995, Abachi et al. 1995, Carithers and Grannis 1995). By 1990, searches by CDF had raised the lower bound on the top-quark mass to 91 GeV, excluding decays of W into t + b. A heavy top decays so swiftly that it cannot be observed directly, but must be inferred from its disintegration into a bottom quark and a W boson – both of which are themselves unstable particles. The hunt took off with the growing data-sets available to both CDF and DØ in 1992–1993 and soon the possibility of observing top was in the air. DØ subsequently raised the lower bound to 131 GeV. Moreover, a growing body of observations that probed quantum corrections to the electroweak theory pointed to a top-quark mass in the range 150–200 GeV. Finding top there emerged as a critical test of the understanding built up over two decades.
Eighteen months of deliciously intense activity culminated in a joint seminar on 2 March 1995, demonstrating that top was found in the reaction pp → tt+ anything. CDF gauged the top-quark mass at 176 ± 13 GeV, while DØ reported 199 ± 30 GeV. Since the discovery, larger event samples, improved detectors and sophisticated analysis techniques have led to a detailed dossier of top-quark properties (Deliot and Glenzinski 2010). Tevatron measurements of the top mass have reached 0.54% precision, at 173.2 ± 0.9 GeV, a level that demands scrupulous attention to the theoretical definition of what is being measured (Tevatron Electroweak Working Group 2011). A compilation of the Tevatron measurements is shown in figure 5. CDF and DØ now aim for an uncertainty of ± 1 GeV per experiment; to reach this level of precision will require a better understanding of b-jet modelling and of uncertainties in the signal and background simulations.

The tt production characteristics are in good agreement with QCD expectations for the total rate, transverse-momentum dependence and invariant-mass distribution. Tevatron studies support a top-quark charge of +2/3, and show that the tbW interaction is left-handed. Approximately 70% of the W bosons emitted in top decay are longitudinally polarized, while the rest are left-handed. The top-quark lifetime is close to 0.3 yoctosecond (10–24 s), as electroweak theory anticipates. Because top decays before hadronizing, it can be studied as a bare quark. Up to this point, exploratory studies of spin correlations among the tt decay products are in accord with the Standard Model. Both experiments have observed a forward-backward production asymmetry that is considerably larger than the Standard Model predictions, as currently understood. This tantalizing result – which could point to new physics – challenges theorists to create more robust, detailed and credible simulations of the Standard Model.
Important information about the weak interactions of top comes from the detection of single-top production through the decay of a virtual W boson or the interaction of an exchanged W boson with a b quark. Using an array of multivariate analysis techniques, CDF and DØ have observed single-top production at a rate consistent with the Standard Model. The DØ collaboration has succeeded in isolating the t-channel exchange process. These measurements allow a determination of the strength of the tbW weak coupling that is consistent with the Standard Model prediction of a value near unity, as well as with other indications that t → bW is the dominant decay mode of the top quark.
Higgs and other new phenomena
The search for the Standard Model Higgs boson is the ultimate challenge for the Tevatron. The straightforward strategy, to detect a light Higgs boson produced in gluon–gluon fusion that decays into the dominant bb mode, is foreclosed by the overwhelming rate of b-quark pair production by the strong interactions. Thus CDF and DØ have had to seek signals in several production channels and decay modes, as well as master many sources of background. Current searches consider gluon–gluon fusion, the associated production of a Higgs boson and W or Z boson and vector-boson fusion. The decay modes examined are bb, W+W–, ZZ, γγ and τ+τ–.
So far, the Tevatron experiments have given information on where the Standard-Model Higgs boson is not. The combined analyses of summer 2011, based on up to 8.6 fb–1 of data, exclude Standard Model Higgs-boson masses between 156 and 177 GeV, as shown in figure 6 (The Tevatron New-Phenomena and Higgs Working Group 2011). Parallel work has restricted the allowed parameter space for the lightest Higgs boson of supersymmetric models. According to projections informed by current experience, the full Tevatron data-set should yield 95% confidence-level exclusion limits up to 185 GeV – should no signal be present – and “evidence” at the 3σ level below 120 GeV and in the range 150–175 GeV.
During more than two decades as the world’s highest-energy machine, the Tevatron has had unparalleled capability to search for direct manifestations of physics beyond the Standard Model. Broad explorations and searches for specific hypothetical phenomena have been major activities for the experiments. The Tevatron constraints on conjectured extensions to the Standard Model are impressive in number and scope: CDF and DØ have set limits on supersymmetric particles, many varieties of extra spatial dimensions, signs of new strong dynamics, carriers of new forces of nature, magnetic monopoles and many more exotica. The null searches compel us to contemplate with greater intensity the unreasonable effectiveness of the Standard Model.
To be sure, some observations do not square with conventional expectations. In addition to the suggestion of a larger-than-foreseen forward-backward asymmetry in top-pair production noted above, it is worth mentioning two other surprising effects now in play. DØ reports an anomalous like-sign dimuon charge asymmetry in semileptonic decays of bb pairs that suggests unexpectedly large CP violation in the decays of b-hadrons. CDF sees a yield of jet pairs in association with a W boson that exceeds expectations in the dijet mass interval between 120 and 160 GeV. DØ does not confirm the excess, but the degree of disagreement remains to be quantified. We should find out soon, from further work at the Tevatron and from new analyses at the LHC, whether any of these results holds up and changes our thinking.