From targets to absorbers, beam-intercepting devices are vital to CERN’s accelerator complex. Marco Calviani describes the major upgrades taking place to prepare for the high-luminosity LHC, and the challenges posed by future projects.
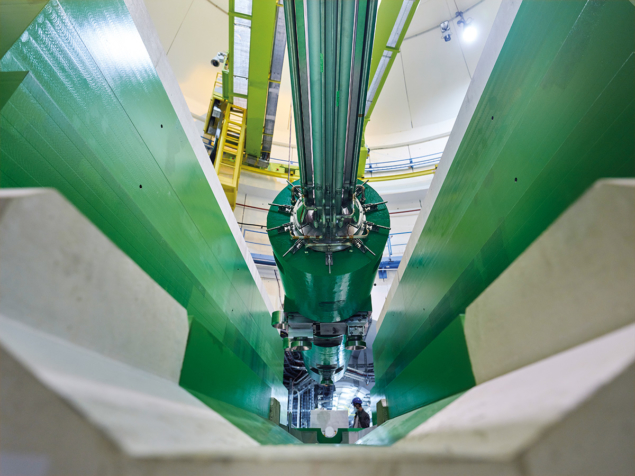
Imagine standing in the LHC tunnel when the machine is operating. Proton beams are circulating around the 27 km ring more than 11,000 times per second, colliding at four points to generate showers of particles that are recorded by ATLAS, CMS, ALICE, LHCb and other detectors. After a few hours of operation, the colliding beams need to be disposed of to allow a new physics fill. Operators in the CERN control centre instruct beam-transfer equipment to shunt the circulating beams into external trajectories that transport them away from the cryogenic superconducting magnets. Each beam exits the ring and travel for 600 metres in a straight line before reaching a compact cavern housing a large steel cylinder roughly 9 m long, 70 cm in diameter and containing about 4.4 tonnes of graphitic material. Huge forces are generated in the impact. If you could witness the event up close, you would hear a massive “bang” – like a bell – generated by the sudden expansion and successive contraction of the steel shell.
What you will have witnessed is a beam-intercepting system in action. Of course, experiencing a beam dump in person is not possible, due to the large amount of radiation generated in the impact, which is one of the reasons why access to high-energy accelerators is strictly forbidden during operation.
Beam-intercepting systems are essential devices designed to absorb the energy and power of a particle beam. Generally, they are classified in three categories depending on their use: particle-producing devices, such as targets; systems for beam cleaning and control, such as collimators or scrapers; and those with safety functions, such as beam dumps or beam stoppers. During the current long-shutdown 2 (LS2), several major projects have been undertaken to upgrade some of the hundreds of beam-intercepting systems across CERN’s accelerator complex, in particular to prepare the laboratory for the high-luminosity LHC era.
Withstanding stress
Beam-intercepting devices have to withstand enormous mechanical and thermally-induced stresses. In the case of the LHC beam dump, for example, upgrades of the LHC injectors will deliver a beam which at high energy will have a kinetic energy equivalent to 560 MJ during LHC Run 3, roughly corresponding to the energy required to melt 2.7 tonnes of copper. Released in a period of just 86 μs, this corresponds to a peak power of 6.3 TW or, put differently, 8.6 billion horse power.

In general, the energy deposited in beam-intercepting devices is directly proportional to the beam energy, its intensity and the beam-spot size, as well as to the density of the absorbing material. From the point of view of materials, this energy is transformed into heat. In a beam dump, for example, the collision volume (which is usually much smaller than the beam-intercepting device itself) is heated to temperatures of 1500 C or more. This heat causes the small volume to try to expand but, because the surrounding area has a much lower temperature, there is no room for expansion. Instead, the hot volume pushes against the colder surrounding area, risking breaking the structure. To reach a sufficient attenuation, due to the high energy of the beams in CERN’s accelerators, we need devices that in some cases are several metres long.
Beam-intercepting devices must be able to withstand routine operation and also accident scenarios, where they serve to protect more delicate equipment such as cryomagnets. Amongst the many challenges that need to be faced are operation under ultra-high-vacuum conditions, and maintaining integrity and functionality when enduring energy densities up to several kJ/cm3 or power densities up to several MW/cm3. For physics applications, optimisation processes have led to the use of low-strength materials, such as pure lead for the generation of neutrons at the n_TOF facility or iridium and tantalum for the generation of antiprotons at the Antiproton Decelerator (AD) facility.
Preparing for HL-LHC
The LHC Injectors Upgrade (LIU) Project, which was launched in 2010 and for which the hardware was installed during LS2, will allow beams with a higher intensity and a smaller spot size to be injected into the LHC. This is a precondition for the full execution of the High-Luminosity LHC (HL-LHC), which will enable a large increase in the integrated luminosity collected by the experiments. To safely protect sensitive equipment in the accelerator chain, the project required a series of new devices in the injector complex from the PS Booster to the SPS, including new beam-intercepting devices. One example is the new SPS internal beam dump, the so-called TIDVG (Target Internal Dump Vertical Graphite), which was installed in straight-section five of the SPS during 2020 (see “Structural integrity” image). The main challenge faced for this device was the need to dissipate a large amount of power from the device rapidly and efficiently to avoid reaching temperatures not acceptable by the beam-dump materials.
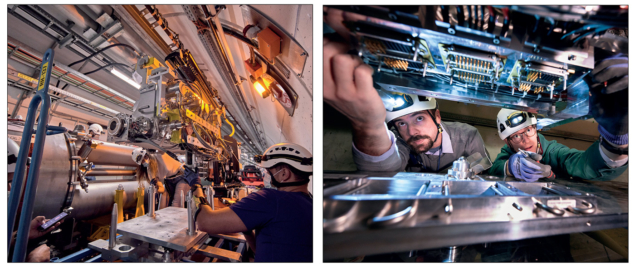
The TIDVG is used to dispose of the SPS circulating beam whenever necessary, for example in case of emergency during LHC beam-setup, filling or machine-development periods, and to dispose of the part of the beam dedicated to fixed-target experiments that remains after the slow-extraction process. Aiming at reducing the energy density deposited in the dump core’s absorbing material (and hence minimising the associated thermo-mechanical stresses), the beam is diluted by kicker magnets, producing a sinusoidal pattern on the front of the first absorbing block. The dump is designed to absorb all beam energies in the SPS, from 14 GeV (injection from the PS) to 450 GeV.
The LHC Injectors Upgrade Project will allow beams with a higher intensity and a smaller spot size to be injected into the LHC
With respect to the pre-LS2 device, the beam power to be absorbed by the dump will be four-times higher, with an average power of 300 kW. To reduce the local energy deposition whilst maintaining the total required beam absorption, the length of the new dump has been increased by 70 cm, leading to a 5 m-long dump. The dump blocks are arranged so that the density of the absorbing materials increases as the beam passes through the device: 4.4 m of isostatic graphite, 20 cm of a molybdenum alloy and 40 cm of pure tungsten. This ensures that the stresses associated with the resulting thermal gradients are kept within acceptable values. The core of the component, which receives the highest thermal load, is cooled directly by a dedicated copper-alloy jacket surrounding the blocks, which can only release their heat through the contact with the jacket; to maximise the thermal conductivity at the interfaces between the stainless-steel cooling pipes and the copper alloy, these materials are diffusion-bonded by means of hot isostatic pressing. The entire core is embedded in an air-cooled, seamless 15 mm-thick stainless-steel hollow cylinder. Due to the high activation of the dump expected after operation, in addition to the first cast-iron shielding, the assembly is surrounded by a massive, multi-layered external shield comprising an inner layer of 50 cm of concrete, followed by 1 m of cast iron and an external layer 40 cm of marble. Marble is used on the three sides accessible by personnel to minimise the residual dose rate in the vicinity after short cool-down times.
Collimator system upgrades
Beam collimators and masks are essential components in accelerator systems. They act as intermediate absorbers and dilutors of the beam in case of beam losses, minimising the thermal energy received by components such as superconducting magnets (leading to quench) or delicate materials in the LHC experiments. The other function of the collimators is to clean up the halo of the beam, by removing particles moving away from the correct orbit. Collimators generally consist of two jaws – moveable blocks of robust materials – that close around the beam to clean it of stray particles. More than 100 of these vital devices are placed around the LHC in critical locations.
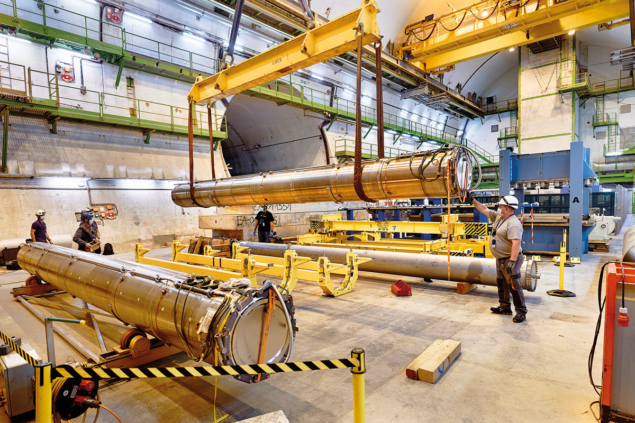
The jaw materials can withstand extreme temperatures and stresses (resulting in deposited energy densities up to 6 kJ/cm3), while maintaining – at least for the LHC collimators – good electrical conductivity to reduce the impedance contribution to the machine. Several developments were incorporated in the SPS-to-LHC transfer line collimators built in the framework of the LIU project, as well as in the LHC collimators for the HL-LHC. For the former, dedicated and extremely robust 3D carbon-composite materials were developed at CERN in collaboration with European industry, while for the latter, dedicated molybdenum carbide-graphite composites were developed, again in collaboration with European firms. For these cases, more than 30 new collimators have been built and installed in the SPS and LHC during LS2 (see “New collimators” image).
LHC beam-dump upgrades
Several challenges associated with the LHC beam dump system had to be overcome, especially on the dump-block itself: it needs to be ready at any time to accept protons, from injection at 450 GeV up to top energy (6.5 TeV, with up to 7 TeV in the future); it must be reliable (~200 dump events per year); and it must accept fast-extracted beams, given that the entire LHC ring is emptied in just 86 μs. At 560 MJ, the projected stored beam energy during Run 3 will also be 75% higher than it was during Run 2.
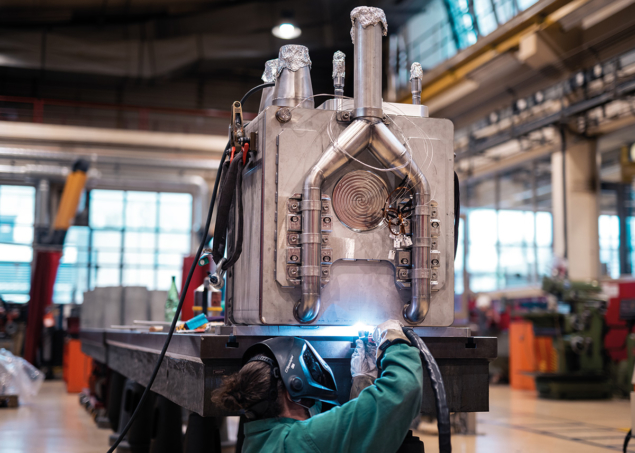
The dump core (around 8 m long) consists of a sandwich of graphitic materials of sufficiently low density to limit the temperature rise – and therefore the resulting thermal-induced stresses – in the material (see “End of the line” image). The graphite is contained in a 12 mm-thick special stainless-steel grade (see “Dump upgrades” image) and the assembly is surrounded by shielding blocks. Roughly 75% (±430 MJ) of the energy that gets deposited by either electromagnetic shower and ionisation losses of hadrons and muons is deposited in the graphite, while around 5% (±25 MJ) is deposited in the thin steel vessel, and the remaining energy is deposited in the shielding assembly. Despite the very low density (1.1 g/cm3) employed in the middle section of the core, temperatures up to 1000 C have been reached during Run 2. From Run 3, temperatures up to 1500 C will be reached. These temperatures could be much higher if it were not for the fact that the beam is “painted” on the face of the dump by means of dilution kickers situated hundreds of metres upstream. The dump must also guarantee its structural integrity even in the case of failures of these dilution systems.
Although the steel vessel is responsible for absorbing just 5% of the deposited energy, the short timescales involved lead to a semi-instantaneous rise in temperature of more than 150 C, generating accelerations up to 2000 g and forces of several hundred tonnes. Following the operational experience during LHC Run 1 and Run 2, during LS2 several upgrades have been implemented on the dump. These include complex instrumentation to yield information and operational feedback during Run 3, until 2025. In the later HL-LHC era, the dump will have to absorb an additional 50% more energy per dump than during Run 3 (up to 750 MJ/dump), presenting one of numerous beam-interception challenges to be faced.
Fixed-target challenges
Beyond the LHC, challenging conditions are also encountered for antiproton production at CERN’s Antiproton Decelerator (AD), which serves several antimatter experiments. In this case, high-density materials are required to make sources as point-like as possible to improve the capture capabilities of the downstream magnetic-horn focusing system. Energy densities up to 7 kJ/cm3 and temperatures up to 2500 C are reached in refractory materials such as iridium, tantalum and tungsten. Such intense energy densities and the large gradients resulting from the very small transverse beam size generate large thermal stresses and produce damage in the target material, which must be minimised to maintain the reliability of the AD’s physics programme. To this end, a new air-cooled antiproton production target will be installed in the antiproton target area this year. Similar challenges are faced when producing neutrons for the n_TOF facility: in this case a new nitrogen-cooled pure lead spallation target weighing roughly 1.5 tonnes will be commissioned this year, ready to produce neutrons spanning 11 orders of magnitude in energy, from 25 meV to several GeV (see “Neutron production target” image).

Reliability is a key aspect in the construction of beam-intercepting devices, not just because machine operation strongly depends on them, but because replacing devices is not easy due to their residual radioactivation after operation. But how do we know that new devices will fulfill their function successfully once installed in the machine? CERN’s HiRadMat facility, which allows single proton pulse testing using a high-intensity beam from the SPS, is one solution. Extremely high energy densities can be reached in test materials and in complex systems, allowing the experimental teams to investigate – in a controlled manner – the behaviour of materials or complex mechanical systems when impacted by proton (or ion) beams. During the past few years, the facility was heavily employed by both CERN and external teams from laboratories such as STFC, Fermilab, KEK and GSI, testing materials from graphite to copper and iridium across the whole spectrum of densities (see “Material integrity test” image). To be able to correctly predict the behaviour of materials when impacted by protons and other charged particles, a full understanding of thermo-physical and material properties is mandatory. Examples of critical properties include the coefficient of thermal expansion, heat capacity, thermal and electrical conductivity as well as the Young’s modulus and yield strength, as well as their temperature dependence.
Dealing with radiation damage is becoming increasingly important as facilities move to higher beam intensities and energies, presenting potential show-stoppers for some beam-intercepting devices. To better understand and predict the radiation response of materials, the RaDIATE collaboration was founded in 2012, bringing together the high-energy physics, nuclear and related communities. The collaboration’s research includes determining the effect of high-energy proton irradiation on the mechanical properties of potential target and beam-window materials, and developing our understanding via micro-structural studies. The goal is to enable accurate lifetime predictions for materials subjected to beam impact, to design robust components for high-intensity beams, and to develop new materials to extend lifetimes. CERN is partner to this collaboration, as well as Fermilab, STFC/UKRI, Oak Ridge, KEK, Pacific Northwest National Laboratory, and other institutions and laboratories worldwide.
Future projects
High-energy physics laboratories across the world are pursuing new energy and/or intensity frontiers, either with hadron or lepton machines. In all cases, whether collider physics or fixed-target, neutrino or beam-dump experiments, beam-intercepting devices are at the heart of accelerator operations. For the proposed 100 km-circumference Future Circular Collider (FCC), several challenges have already been identified. Owing to the small emittances and high luminosities involved in a first electron–positron FCC phase, the positron source system, and its target and capture system, will require dedicated R&D and testing as well as the two lepton dumps. FCC’s proton–proton phase, further in the future, will draw on lessons from the HL-LHC operation, but it will also operate at uncharted energy densities for beam-intercepting devices, both for beam cleaning and shaping collimators as well as for the beam dumps.
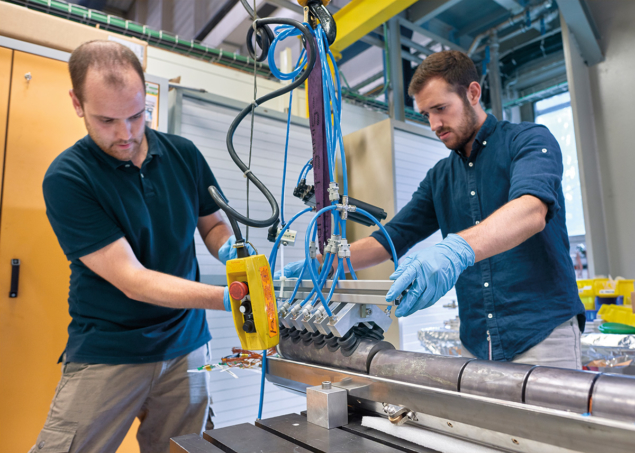
The recently launched muon-collider initiative, meanwhile, will require a target system capable of providing copious amounts of muons generated either by proton beams or electrons impacting on a target, depending on the scheme under consideration. For the former, beams of several MW could collide on a production target, which will have to be very efficient to produce muons of the required momenta while being sufficiently reliable to operate without failure for long periods. The muon collider target and front-end systems will also require magnets and shielding to be located quite close to the production target and will have to cope with radiation load and heat deposition. These challenges will be tackled extensively in the next few years, both from a physics and engineering perspective.
Successful beam-intercepting devices require extensive knowledge and skills
As one of the front-runner projects in the Physics Beyond Colliders initiative, the proposed Beam Dump Facility at CERN would require the construction of a general-purpose high-intensity and high-energy fixed-target complex, initially foreseen to be exploited by the Search for Hidden Particles (SHiP) experiment. At the heart of the installation resides a target/dump assembly that can safely absorb the full high-intensity 400 GeV/c SPS beam, while maximising the production of charm and beauty mesons and using high-Z materials, such as pure tungsten and molybdenum alloy, to reduce muon background for the downstream experiment. The nature of the beam pulse induces very high temperature excursions between pulses (up to 100 °C), leading to considerable thermally induced stresses and long-term fatigue considerations. The high average power deposited on target (305 kW) also creates a challenge for heat removal. A prototype target was built and tested at the end of 2018, at one tenth of the nominal power but able to reach the equivalent energy densities and thermal stresses (see “Beam-dump facility” image).
Human efforts
The development, construction and operation of successful beam-intercepting devices require extensive knowledge and skills, ranging from mechanical and nuclear engineering, to physics, vacuum technologies and advanced production techniques. Technicians also constitute the backbone of the design, assembly and installation of such equipment. International exchanges with experts in the fields and with laboratories working with similar challenges is essential, as is cross-discipline collaboration, for example in aerospace, nuclear and advanced materials. In addition, universities provide key students and personnel capable of mastering and developing these techniques both at CERN and in CERN’s member states’ laboratories and industries. This intense multidisciplinary effort is vital to successfully tackle the challenges related to current and future high-energy and high-intensity facilities and infrastructures, as well as to develop systems with broader societal impact, for example in X-ray synchrotrons, medical linacs, and the production of radioisotopes for nuclear medicine.