Over time, an increasing number of particle physicists became interested in the problem of dark matter, partly driven by new scientific results but also by sociological changes.
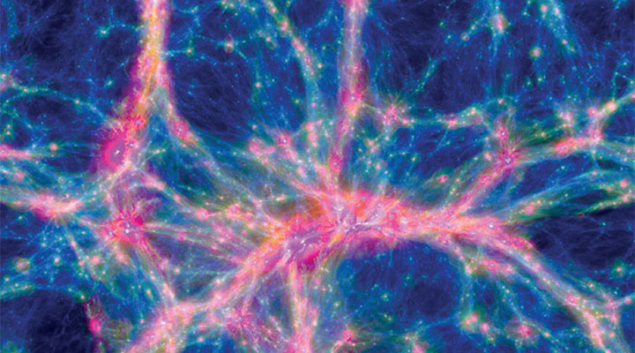
Astronomers have long contemplated the possibility that there may be forms of matter in the universe that are imperceptible, either because they are too far away, too dim or intrinsically invisible. Lord Kelvin was perhaps the first, in 1904, to attempt a dynamical estimate of the amount of dark matter in the universe. His argument was simple yet powerful: if stars in the Milky Way can be described as a gas of particles acting under the influence of gravity, one can establish a relationship between the size of the system and the velocity dispersion of the stars. Henri Poincaré was impressed by Kelvin’s results, and in 1906 he argued that since the velocity dispersion predicted in Kelvin’s estimate is of the same order of magnitude as that observed, “there is no dark matter, or at least not so much as there is of shining matter”.
The Swiss–US astronomer Fritz Zwicky is arguably the most famous and widely cited pioneer in the field of dark matter. In 1933, he studied the redshifts of various galaxy clusters and noticed a large scatter in the apparent velocities of eight galaxies within the Coma Cluster. Zwicky applied the so-called virial theorem – which establishes a relationship between the kinetic and potential energies of a system of particles – to estimate the cluster’s mass. In contrast to what would be expected from a structure of this scale – a velocity dispersion of around 80 km/s – the observed average velocity dispersion along the line of sight was approximately 1000 km/s. From this comparison, Zwicky concluded: “If this would be confirmed, we would get the surprising result that dark matter is present in much greater amount than luminous matter.”
In the 1950s and 1960s, most astronomers did not ask whether the universe had a significant abundance of invisible or missing mass. Although observations from this era would later be seen as evidence for dark matter, back then there was no consensus that the observations required much, or even any, such hidden material, and certainly there was not yet any sense of crisis in the field. It was in 1970 that the first explicit statements began to appear arguing that additional mass was needed in the outer parts of some galaxies, based on comparisons between predicted and measured rotation curves. The appendix of a seminal paper published by Ken Freeman in 1970, prompted by discussions with radio-astronomer Mort Roberts, concluded that: “If [the data] are correct, then there must be in these galaxies additional matter which is undetected, either optically or at 21 cm. Its mass must be at least as large as the mass of the detected galaxy, and its distribution must be quite different from the exponential distribution which holds for the optical galaxy.” (Figure 1 below.)
Several other lines of evidence began to appear that supported the same conclusion. In 1974, two influential papers (by Jaan Einasto, Ants Kaasik and Enn Saar, and by Jerry Ostriker, Jim Peebles and Amos Yahil) argued that a common solution existed for the mass discrepancies observed in clusters and in galaxies, and made the strong claim that the mass of galaxies had been until then underestimated by a factor of about 10.
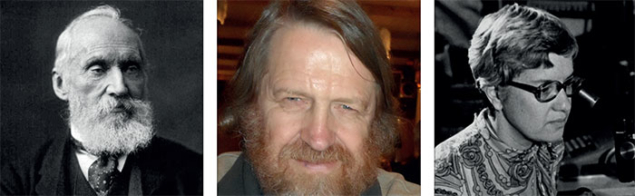
Image credits: (from left) Wikicommons; L Athanassoula ;Carnegie Institution for Science.
By the end of the decade, opinion among many cosmologists and astronomers had crystallised: dark matter was indeed abundant in the universe. Although the same conclusion was reached by many groups of scientists with different subcultures and disciples, many individuals found different lines of evidence to be compelling during this period. Some astronomers were largely persuaded by new and more reliable measurements of rotation curves, such as those by Albert Bosma, Vera Rubin and others. Others were swayed by observations of galaxy clusters, arguments pertaining to the stability of disc galaxies, or even cosmological considerations. Despite disagreements regarding the strengths and weaknesses of these various observations and arguments, a consensus nonetheless began to emerge by the end of the 1970s in favour of dark-matter’s existence.
Enter the particle physicists
From our contemporary perspective, it can be easy to imagine that scientists in the 1970s had in mind halos of weakly interacting particles when they thought about dark matter. In reality, they did not. Instead, most astronomers had much less exotic ideas in the form of comparatively low-luminosity versions of otherwise ordinary stars and gas. Over time, however, an increasing number of particle physicists became aware of and interested in the problem of dark matter. This transformation was not just driven by new scientific results, but also by sociological changes in science that had been taking place for some time.
Half a century ago, cosmology was widely viewed as something of a fringe science, with little predictive power or testability. Particle physicists and astrophysicists did not often study or pursue research in each other’s fields, and it was not obvious what their respective communities might have to offer one another. More than any other problem in science, it was dark matter that brought particle physicists and astronomers together.
As astrophysical alternatives were gradually ruled out one by one, the view that dark matter is likely to consist of one or more yet undiscovered species of subatomic particle came to be held almost universally among both particle physicists and astrophysicists alike.
Perhaps unsurprisingly, the first widely studied particle dark-matter candidates were neutrinos. Unlike all other known particle species, neutrinos are stable and do not experience electromagnetic or strong interactions – which are essential characteristics for almost any viable dark-matter candidate. The earliest discussion of the role of neutrinos in cosmology appeared in a 1966 paper by Soviet physicists Gershtein and Zeldovich, and several years later the topic began to appear in the West, beginning in 1972 with a paper by Ram Cowsik and J McClelland. Despite the very interesting and important results of these and other papers, it is notable that most of them did not address or even acknowledge the possibility that neutrinos could account for the missing mass that had been observed by astronomers on galactic and cluster scales. An exception included the 1977 paper by Lee and Weinberg, whose final sentence reads: “Of course, if a stable heavy neutral lepton were discovered with a mass of order 1–15 GeV, the gravitational field of these heavy neutrinos would provide a plausible mechanism for closing the universe.”
While this is still a long way from acknowledging the dynamical evidence for dark matter, it was an indication that physicists were beginning to realise that weakly interacting particles could be very abundant in our universe, and may have had an observable impact on its evolution. In 1980, the possibility that neutrinos might make up the dark matter received a considerable boost when a group studying tritium beta decay reported that they had measured the mass of the electron antineutrino to be approximately 30 eV – similar to the value needed for neutrinos to account for the majority of dark matter. Although this “discovery” was eventually refuted, it motivated many particle physicists to consider the cosmological implications of their research.
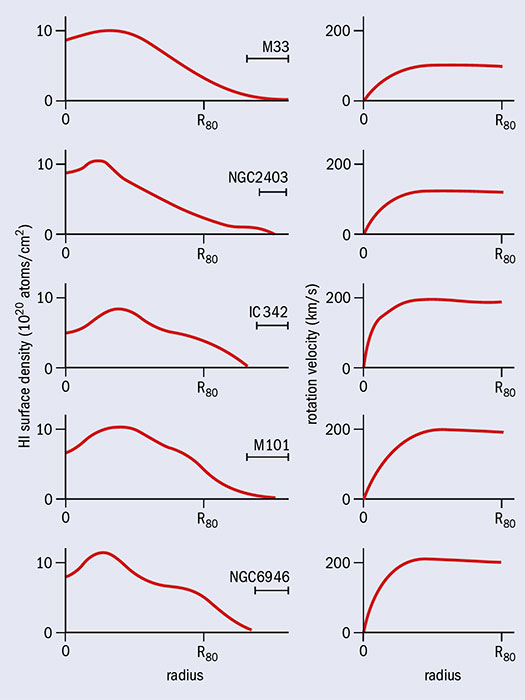
Although we know today that dark matter in the form of Standard Model neutrinos would be unable to account for the observed large-scale structure of the universe, neutrinos provided an important template for the class of hypothetical species that would later be known as weakly interacting massive particles (WIMPs). Astrophysicists and particle physicists alike began to experiment with a variety of other, more viable, dark-matter candidates.
Cold dark-matter paradigm
The idea of neutrino dark matter was killed off in the mid-1980s with the arrival of numerical simulations. These could predict how large numbers of dark-matter particles would evolve under the force of gravity in an expanding universe, and therefore allow astronomers to assess the impact of dark matter on the formation of large-scale structure. In fact, by comparing the results of these simulations with those of galaxy surveys, it was soon realised that no relativistic particle could account for dark matter. Instead, the paradigm of cold dark matter – i.e. made of particles that were non-relativistic at the epoch of structure formation – was well on its way to becoming firmly established.
Meanwhile, in 1982, Jim Peebles pointed out that the observed characteristics of the cosmic microwave background (CMB) also seemed to require the existence of dark matter. If just baryons existed, then one could only explain the observed degree of large-scale structure if the universe started in a fairly anisotropic or “clumpy” state. But by this time, the available data already set an upper limit on CMB anisotropies at a level of 10–4 – too meagre to account for the universe’s structure. Peebles argued that this problem would be relieved if the universe was instead dominated by massive weakly interacting particles whose density fluctuations begin to grow prior to the decoupling of matter and radiation during which the CMB was born. Among other papers, this received enormous attention within the scientific community and helped establish cold dark matter as the leading paradigm to describe the structure and evolution of the universe at all scales.
Solutions beyond the Standard Model
Neutrinos might be the only known particles that are stable, electrically neutral and not strongly interacting, but the imagination of particle physicists did not remain confined to the Standard Model for long. Instead, papers started to appear that openly contemplated many speculative and yet undiscovered particles that might account for dark matter. In particular, particle physicists began to find new candidates for dark matter within the framework of a newly proposed space–time symmetry called supersymmetry. The cosmological implications of supersymmetry were discussed as early as the late 1970s. In Piet Hut’s 1977 paper on the cosmological constraints on the masses of neutrinos, he wrote that the dark-matter argument was not limited to neutrinos or even to weakly interacting particles. The abstract of his paper mentions another possibility made within the context of the supersymmetric partner of the graviton, the spin-3/2 gravitino: “Similar, but much more severe, restrictions follow for particles that interact only gravitationally. This seems of importance with respect to supersymmetric theories,” wrote Hut.
In their 1982 paper, Heinz Pagels and Joel Primack also considered the cosmological implications of gravitinos. But unlike Hut’s paper, or the other preceding papers that had discussed neutrinos as a cosmological relic, Pagels and Primack were keenly aware of the dark-matter problem and explicitly proposed that gravitinos could provide the solution by making up the missing mass. In many ways, their paper reads like a modern manuscript on supersymmetric dark matter, motivating supersymmetry by its various attractive features and then discussing both the missing mass in galaxies and the role that dark matter could play in the formation of large-scale structure. Around the same time, supersymmetry was being further developed into its more modern form, leading to the introduction of R-parity and constructions such as the minimal supersymmetric standard model (MSSM). Such supersymmetric models included not only the gravitino as a dark-matter candidate, but also neutralinos – electrically neutral mixtures of the superpartners of the photon, Z and Higgs bosons.
Over the past 35 years, neutralinos have remained the single most studied candidate for dark matter and have been the subject of many thousand scientific publications. Papers discussing the cosmological implications of stable neutralinos began to appear in 1983. In the first two of these, Weinberg and Haim Goldberg independently discussed the case of a photino (a neutralino whose composition is dominated by the superpartner of the photon) and derived a lower bound of 1.8 GeV on its mass by requiring that the density of such particles does not overclose the universe. A few months later, a longer paper by John Ellis and colleagues considered a wider range of neutralinos as cosmological relics. In Goldberg’s paper there is no mention of the phrase “dark matter” or of any missing mass problem, and Ellis et al. took a largely similar approach by simply requiring only that the cosmological abundance of neutralinos not be so large as to overly slow or reverse the universe’s expansion rate. Although most of the papers on stable cosmological relics written around this time did not yet fully embrace the need to solve the dark-matter problem, occasional sentences could be found that reflected the gradual emergence of a new perspective.
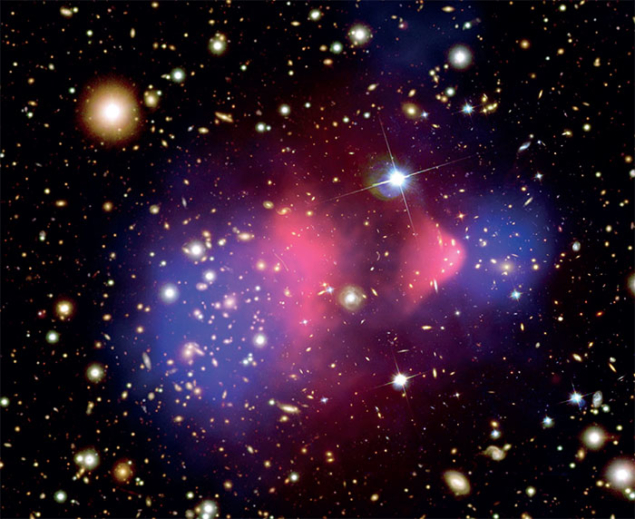
Image credit: NASA/CXC/CfA/ M Markevitch et al.; STScI/ESO WFI; Magellan/D Clowe et al.
During the years that followed, an increasing number of particle physicists would further motivate proposals for physics beyond the Standard Model by showing that their theories could account for the universe’s dark matter. In 1983, for instance, John Preskill, Mark Wise and Frank Wilczek showed that the axion, originally proposed to solve the strong CP problem in quantum chromodynamics, could account for all of the dark matter in the universe. In 1993, Scott Dodelson and Lawrence Widrow proposed a scenario in which an additional, sterile neutrino species that did not experience electroweak interactions could be produced in the early universe and realistically make up the dark matter. Both the axion and the sterile neutrino are still considered as well-motivated dark-matter candidates, and are actively searched for with a variety of particle and astroparticle experiments.
The triumph of particle dark matter
In the early 1980s there was still nothing resembling a consensus about whether dark matter was made of particles at all, with other possibilities including planets, brown dwarfs, red dwarfs, white dwarfs, neutron stars and black holes. Kim Griest would later coin the term “MACHOs” – short for massive astrophysical compact halo objects – to denote this class of dark-matter candidates, in response to the alternative of WIMPs. There is a consensus today, based on searches using gravitational microlensing surveys and determinations of the cosmic baryon density based on measurements of the primordial light-element abundances and the CMB, that MACHOs do not constitute a large fraction of the dark matter.
An alternative explanation for particle dark matter is to assume that there is no dark matter in the first place, and that instead our theory of gravity needs to be modified. This simple idea, which was put forward in 1982 by Mordehai Milgrom, is known as modified Newtonian dynamics (MOND) and has far-reaching consequences. At the heart of MOND is the suggestion that the force due to gravity does not obey Newton’s second law, F = ma. If instead gravity scaled as F = ma2/a0 in the limit of very low accelerations (a << a0 ~ 1.2 × 10−10 m/s2), then it would be possible to account for the observed motions of stars and gas within galaxies without postulating the presence of any dark matter.
In 2006, a group of astronomers including Douglas Clowe transformed the debate between dark matter and MOND with the publication of an article entitled: “A direct empirical proof of the existence of dark matter”. In this paper, the authors described the observations of a pair of merging clusters collectively known as the Bullet Cluster (image above left). As a result of the clusters’ recent collision, the distribution of stars and galaxies is spatially separated from the hot X-ray-emitting gas (which constitutes the majority of the baryonic mass in this system). A comparison of the weak lensing and X-ray maps of the bullet cluster clearly reveals that the mass in this system does not trace the distribution of baryons. Another source of gravitational potential, such as that provided by dark matter, must instead dominate the mass of this system.
Following these observations of the bullet cluster and similar systems, many researchers expected that this would effectively bring the MOND hypothesis to an end. This did not happen, although the bullet cluster and other increasingly precise cosmological measurements on the scale of galaxy clusters, as well as the observed properties of the CMB, have been difficult to reconcile with all proposed versions of MOND. It is currently unclear whether other theories of modified gravity, in some yet-unknown form, might be compatible with these observations. Until we have a conclusive detection of dark-matter particles, however, the possibility that dark matter is a manifestation of a new theory of gravity remains open.
Today, the idea that most of the mass in the universe is made up of cold and non-baryonic particles is not only the leading paradigm, but is largely accepted among astrophysicists and particle physicists alike. Although dark-matter’s particle nature continues to elude us, a rich and active experimental programme is striving to detect and characterise dark-matter’s non-gravitational interactions, ultimately allowing us to learn the identity of this mysterious substance. It has been more than a century since the first pioneering attempts to measure the amount of dark matter in the universe. Perhaps it will not be too many more years before we come to understand what that matter is.