Thanks to its extraordinary radiopurity, Borexino has definitively observed the two main fusion reactions in stars and will soon weigh in on a controversy relating to the birth of the Sun that challenges the basic assumptions of the Solar Standard Model, write Gianpaolo Bellini and Aldo Ianni.

Each second, fusion reactions in the Sun’s core fling approximately 60 billion neutrinos onto every square centimetre of the Earth. In the late 1990s, the Borexino experiment at Gran Sasso National Laboratory in Italy was conceived to measure these neutrinos right down to a few tens of keV, where the bulk of the flux lies. The detector’s name means “little Borex” and refers to an earlier idea for a large experiment with a boron-loaded liquid scintillator, which was shelved in favour of the present, smaller and more ambitious detector. Rather than studying rare but high-energy 8B neutrinos from a little-followed branch of the proton–proton (pp) fusion chain, Borexino would target the far more numerous but lower energy neutrinos produced in the Sun by electron captures on 7Be.

Three decades after its conception, Borexino has far exceeded this goal thanks to the exceptional radiopurity of the experimental apparatus (see “Detector design” panel. Special care taken in construction and commissioning has achieved a radiopurity about three orders of magnitude better than predicted, and 10 to 12 orders of magnitude below natural radioactivity. This has allowed the collaboration to probe the entire solar-neutrino spectrum, including not only the pp chain, but also the carbon–nitrogen–oxygen (CNO) cycle. This mechanism plays a minor role in the Sun but becomes important for more massive stars, dominating the energy production and the production of elements heavier than helium in the universe at large.
The heart of the Sun
The pp-chain generates 99% of the energy in the Sun: it begins when two protons fuse to produce a deuteron and an electron neutrino – the so-called pp neutrino (see “Chain and cycle” figure). Subsequent reactions produce light elements, such as 3He, 4He, 7Be, 7Li, 8B and more electron neutrinos. In Borexino, the sensitivity to pp neutrinos depends on the amount of 14C in the liquid scintillator: with an end-point energy of 0.156 MeV compared with a maximum visible energy for pp neutrinos of 0.264 MeV, the 14C → 14N + β– + ν beta decay sets the detection threshold and the feasibility of probing pp-neutrinos. The Borexino scintillator was therefore made using petroleum from very old and deep geological layers, to ensure a low content of 14C.
Detector design
Like many particle-physics detectors, Borexino has an onion-like design. The innermost layers have the highest radio-purity. The detector’s active core consists of 278 tonnes of pseudocumene (C9H12) scintillator. Into this is dissolved 2,5-diphenyloxazole (PPO) at a concentration of 1.5 grams per litre, which shifts the emission light to 400 nm, where the sensitivity of photomultipliers is peaked. The scintillator is contained within a 125 μm-thick nylon inner vessel (IV) with a 4.5 m radius – made thin to reduce radiation emission from the nylon . In addition, the IV stops radon diffusion towards the core of the detector.
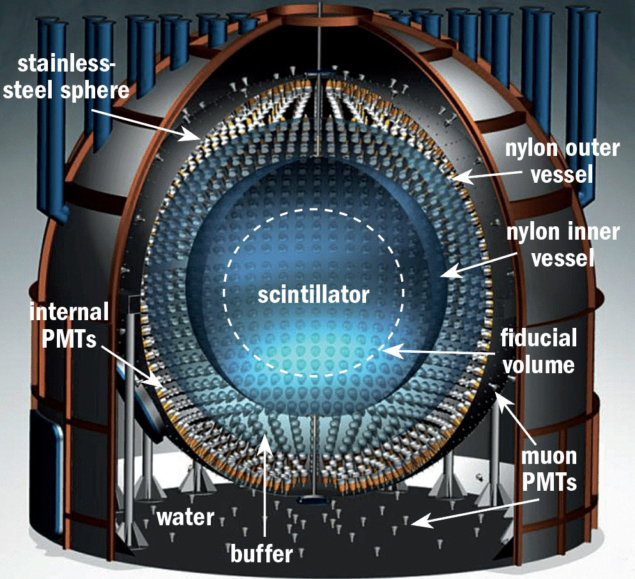
The IV is contained within a 7 m-radius stainless-steel sphere (SSS) that supports 2212 photomultipliers (PMTs) and contains 1000 tonnes of pseudocumene as high-radio-purity shielding liquid against radioactivity from PMTs and the SSS itself. Between the SSS and the IV, a second nylon balloon acts as a barrier preventing radon and its progeny from reaching the scintillator. The SSS is contained in a 2400-tonne tank of highly purified water which, together with Borexino’s underground location, shields the detector from environmental radioactivity. The tank boasts a muon detector to tag particles crossing the detector.
When a neutrino interacts in the target volume, energy deposited by the decelerating electron is registered by a handful of PMTs. The neutrino’s energy can be obtained from the total charge, and the hit-time distribution is used to infer the location of the event’s vertex. Recoiling electrons are used to tag electron neutrinos, and the combination of a positron annihilation and a neutron capture on hydrogen (an inverse beta decay) are used to tag electron antineutrinos.
Due to the impossibility of discriminating individual solar-neutrino events from the backgrounds, the greatest challenge has been the reduction of natural radioactivity to unprecedented levels. In the early 1990s, Borexino developed innovative techniques such as under-vacuum distillation, water extraction, ultrafiltration and nitrogen sparging with ultra-high radiopurity nitrogen to reduce radioactive impurities in the scintillator to 10–10 Bq/kg or better. An initial detector called the Counting Test Facility was developed as a means to demonstrate such claims, publishing results for the key uranium, thorium and krypton backgrounds in 1995. Full data taking at Borexino began in 2007.
Since data-taking began in 2007, Borexino has measured, for the first time, all the individual fluxes produced in the pp-chain. In 2014 the collaboration made the first definitive observation of pp neutrinos, using a comparison with the predicted energy spectrum. In 2018 the collaboration performed, with the same apparatus, a measurement of all the pp-chain components (pp, 7Be, pep and 8B neutrinos), demonstrating the large-scale energy-generation mechanism in the Sun for the first time (see “Energy spectrum” figure). This spectral fit allowed the collaboration to directly determine the ratio between the interaction rate of 3He + 3He fusions and that of 3He + 4He fusions – a crucial parameter for characterising the pp chain and its energy production.
The simultaneous measurement of pp-chain neutrino fluxes also gave Borexino a unique window onto the famous “vacuum-matter” transition, whereby coherent virtual W-boson interactions with electrons modify neutrino-oscillation probabilities as neutrinos propagate through matter, enhancing the oscillation probability as a function of energy. In 2018 Borexino measured the solar electron–neutrino survival probability, Pee, in the energy range from a few tens of keV up to 15 MeV (see “Survival probability” figure). This was the first direct observation of the transition from a low-energy vacuum regime (Pee~0.55) to a higher energy matter regime where neutrino propagation is dominantly affected by the solar interior (Pee~0.32). The transition was measured by Borexino at the level of 98% confidence.
CNO cycle
A different way to burn hydrogen, the CNO cycle, was hypothesised independently by Carl Friedrich von Weizsäcker and Hans Albrecht Bethe between 1937 and 1939. Here, 12C acts as a catalyst, and electron neutrinos are produced by the beta decay of 13N and 15O, with a small contribution from 17F. The maximum energy of CNO neutrinos is about 1.7 MeV. In addition to making an important contribution to the production of elements heavier than helium, this cycle is important for the nucleosynthesis of 16O and 17O. In massive stars it also develops in more complex reactions producing 18F, 18O, 19F, 18Ne and 20Ne.

The sensitivity to CNO neutrinos in Borexino mainly comes from events in the energy range from 0.8 to 1 MeV. In this region, the dominant background comes from 210Bi, which is produced by the slow radioactive decay 210Pb (22 y) → 210Bi (5 d) + β– + ν → 210Po (138 d) + β– + ν → 206Pb (stable) + α. The 210Bi activity can be inferred from 210Po, which can be efficiently tagged using pulse-shape discrimination. However, convective currents in the liquid scintillator bring into the central fiducial mass 210Po produced by 210Pb, which is most likely to be embedded on the nylon containment vessel. In order to reduce convection currents, a passive insulation system and a temperature control system were installed in 2016, significantly reducing the effect of seasonal temperature variations.
Thanks to these and other efforts, in 2020 Borexino rejected the null hypothesis of no CNO reactions by more than five standard deviations, providing the first direct proof of the process. The energy production as a fraction of the solar luminosity was measured to be 1-0.3+0.4 %, in agreement with the Solar Standard Model (SSM) prediction of roughly 0.6 ± 0.1% (which assumes the solar surface has a high metallicity – a topic discussed in more detail later). Given that luminosity scales as M4 and number density as M–2.5 for stars between one and 10 solar masses, the CNO cycle is thought to be the most important source of energy in massive hydrogen-burning stars. Borexino has provided the first experimental evidence for this hypothesis.
Probing solar metallicity using CNO neutrinos is of the utmost importance, and Borexino is hard at work on the problem
But, returning to the confines of our solar system, it’s important to remember that the SSM is not a closed book. Borexino’s results are thus far in agreement with its assumption of a protostar that had a uniform composition throughout its entire volume when fusion began (“zero-age homogeneity”). However, thanks to the ability of neutrinos to peek into the heart of the Sun, the experiment now has the potential to explore this assumption and weigh in on one of the most intriguing controversies in astrophysics.
The solar-abundance controversy
As stars evolve, the distribution of elements within them changes thanks to fusion reactions and convection currents. But the composition of the surface is thought to remain very nearly the same as that of the protostar, as it is not hot enough there for fusion to occur. Measuring the abundance of elements on a star’s surface therefore gives an idea of the protostar’s composition and is a powerful way to constrain the SSM.

Currently, the best method to determine the surface abundance of elements heavier than helium (“metallicity”) uses measurements of photo-absorption lines. Since 2005, improved hydrodynamic calculations (which are needed to model atomic-line formation, and radiative and collisional processes which contribute to excitation and ionisation) indicate a much lower surface metallicity than was previously considered. However, helioseismology observables differ by roughly five standard deviations from SSM predictions that use the new surface metallicity to infer the protostar’s composition, when the sound–speed profile, surface–helium abundance and the depth of the convective envelope are taken into account. Helioseismology implies that the zero-age Sun’s core was richer in metallicity than the present surface composition, suggesting a violation of zero-age homogeneity and a break with the SSM. This is the solar-abundance controversy, which was discovered in 2005.
One possible explanation is that a late “dilution” of the Sun’s convective zone occurred due to a deposition of elements during the formation of the solar system. Were there to have been an accretion of dust and gas from the proto-planetary disc onto the central star during the evolution of the star–planet system, this could have changed the initial metallicity of the surface of the Sun – a hypothesis backed up by recent simulations that show that a metal-poor accretion could produce the present surface metallicity.
As they are an excellent probe of metallicity, CNO neutrinos have an important role to play in settling the solar-abundance controversy. If Borexino were to measure the Sun’s present core metallicity, and by running simulations backwards prove that its surface metallicity must have been diluted right from its birth, this would violate one of the basic assumptions of the SSM. Probing solar metallicity using CNO neutrinos is, therefore, of the utmost importance, and Borexino is hard at work on the problem. Initial results favour the high-metallicity hypothesis with a significance of 2.1 standard deviations – a tentative first hint from Borexino that zero-age homogeneity may indeed be false.
The ancient question of why and how the Sun and stars shine finally has a comprehensive answer from Borexino, which has succeeded thanks to the detector’s extreme and unprecedented radio-purity – the hard work of hundreds of researchers over almost three decades.
Further reading
Borexino collaboration 2020 Nature 587 577.
Borexino collaboration 2020 Phys. Rev. D 101 062001.
Borexino collaboration 2019 Phys. Rev. D 100 082004.
Borexino collaboration 2018 Nature 562 505.
R Hoppe et al. 2020 A&A 641 A73.