Philip Bryant and Kurt Hübner describe the pioneering ISR.

The concept of a particle collider was first laid down by Rolf Widerøe in a German patent that was registered in 1943 but not published until 1952. It proposed storing beams and allowing them to collide repeatedly so as to attain a high energy in the centre-of-mass. By 1956, the first ideas for a realistic proton–proton collider were being publicly discussed, in particular by Donald Kerst and Gerard O’Neill. At the end of the same year, while CERN’s Proton Synchrotron (PS) was still under construction, the CERN Council set up the Accelerator Research Group, which from 1960 onwards focused on a proton–proton collider. By 1962, the group had chosen an intersecting ring layout for the collider over the original concept of two tangential rings because it offered more collision points. Meanwhile, in 1961, CERN had been asked by Council to include a 300 GeV proton synchrotron in the study.
In 1960 construction began on a small proof-of-principle 1.9 MeV electron storage ring, the CERN Electron Storage and Accumulation Ring (CESAR). This was for experimental studies of particle accumulation (stacking). This concept, which had been proposed by the Midwestern Universities Research Association (MURA) in the US in 1956, would be essential for obtaining sufficient beam current and, in turn, luminosity. The design study for the Intersecting Storage Rings (ISR) was published in 1964 – involving two interlaced proton-synchrotron rings that crossed at eight points.
Those who were against the ISR were afraid of the leap in accelerator physics and technology required by this venture
After an intense and sometimes heated debate, Council approved the principle of a supplementary programme for the ISR at its meeting in June 1965. The debate was between those who favoured a facility to peep at interactions at the highest energies and those who preferred intense secondary beams with energies higher than that provided by the PS. Those who were against the ISR were also afraid of the leap in accelerator physics and technology required by this venture, which appeared to them as a shot into the dark.

France made land available for the necessary extension to the CERN laboratory and the relevant agreement was signed in September 1965. The funds for the ISR were allocated at the Council meeting in December of the same year and Kjell Johnsen was appointed project leader. Finally, Greece was the only country out of the 14 member states whose budget did not allow it to participate in the construction. In parallel, the study of a 300 GeV proton synchrotron was to be continued; this would eventually lead to the construction of the Super Proton Synchrotron (SPS) at CERN.
Figure 1 shows the ISR layout with the two rings intersecting at eight points at an angle of 15°. To create space for straight sections and to keep the intersection regions free of bulky accelerator equipment, the circumference of each ring was set at 943 m, or 1.5 times that of the PS. Out of the eight intersection regions (I1–I8) six were available for experiments and two were reserved for operation (I3 for beam dumping and I5 for luminosity monitoring).
The ISR construction schedule benefited from the fact that the project had already been studied for several years and many of the leading staff had been involved in the construction of the PS. The ground-breaking ceremony took place in autumn 1966 and civil engineering started for the 15-m wide and 6.5-m high tunnel, using the cut-and-fill method at a level 12 m above the PS to minimize excavation. The construction work also included two large experimental halls (I1 and I4) and two transfer tunnels from the PS to inject the counter-rotating beams. In parallel, the West Hall was built for fixed-target physics with PS beams; ready in July 1968, it was used for assembling the ISR magnets. The civil engineering for the ISR rings was completed in July 1970, including the earth shielding. The production of the magnet steel started in May 1967 and all of the major components for the rings had been ordered by the end of 1967. The first series magnets arrived in summer 1968 and the last magnet unit was installed in May 1970.
Pioneering performance
The first proton beam was injected and immediately circulated in October 1970 in Ring 1. Once Ring 2 was available, the first collisions occurred on 27 January 1971 at a beam momentum of 15 GeV/c (figure 2, p27). By May, collisions had taken place at 26.5 GeV/c per beam – the maximum momentum provided by the PS – which was equivalent to protons of 1500 GeV hitting a fixed target.
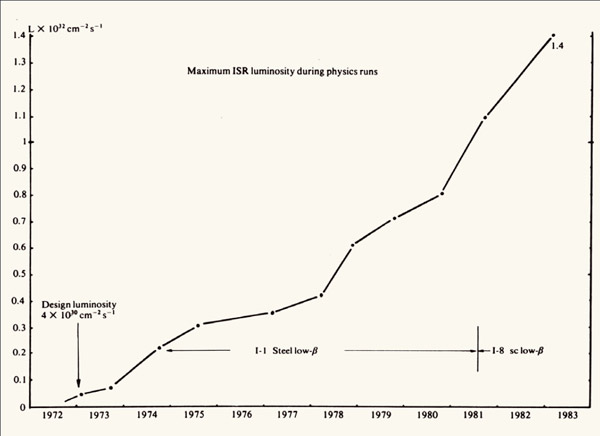
In the first year of operation, the maximum circulating current was already 10 A, the luminosity was as high as 3×1029 cm–2 s–1 and the loss-rates at beam currents of up to 6 A were less then 1% per hour (compared with a design loss-rate of 50% in 12 h). Happily, potentially catastrophic predictions that the beams would grow inexorably and be lost – because, unlike in electron machines, the stabilizing influence of synchrotron radiation would be absent – proved to be unfounded.
The stacking in momentum space, pioneered by MURA, was an essential technique for accumulating the intense beams. In this scheme, the beam from the PS was slightly accelerated by the RF system in the ISR and the first pulse deposited at the highest acceptable momentum on an outer orbit in the relatively wide vacuum chamber. Subsequent pulses were added until the vacuum chamber was filled up to an orbit close to the injection orbit, which was on the inside of the chamber. This technique was essential for the ISR and had been proved experimentally to work efficiently in CESAR.
Borrowed quadrupoles from the PS, DESY and the Rutherford Appleton Laboratory increased the luminosity by a factor of 2.3
The design luminosity was achieved within two years of start-up and then increased steadily, as figure 3 shows. It was particularly boosted in I1 (originally for one year in I7) and later in I8 by low-beta insertions that decreased the vertical size of the colliding beams. The first low-beta insertion, which consisted largely of borrowed quadrupoles from the PS, DESY and the Rutherford Appleton Laboratory, increased the luminosity by a factor of 2.3. Later, for the second intersection, more powerful superconducting quadrupoles were developed at CERN but built by industry. This increased the luminosity by a factor of 6.5, resulting in a maximum luminosity of 1.4×1032 cm–2 s–1. This remained a world record until 1991, when it was broken by the Cornell electron–positron storage ring.
The stored currents in physics runs were 30–40 A (compared with 20 A nominal); the maximum proton current that was ever stored was 57 A. Despite these high currents, the loss rates during physics runs were typically kept to one part per million per minute, which provided the desired low background environment for the experiments. Beams of physics quality could last 40–50 hours.
Because the ISR’s magnet system had a significant reserve, the beams in the two rings could be accelerated to 31.4 GeV/c by phase displacement, a technique that was also proposed by MURA. This consisted of moving empty buckets repeatedly through the stacked beam. The buckets were created at an energy higher than the most energetic stored particles and moved through the stack to the injection orbit. In accordance with longitudinal phase-space conservation, the whole stack was accelerated and the magnet field was simultaneously increased to keep the stack centred in the vacuum chamber. This novel acceleration technique required the development of a low-noise RF system operating at a low voltage with a fine control of the high-stability, magnet power supplies.
The ISR was also able to store deuterons and alpha particles as soon as they became available from the PS, leading to a number of runs with p–d, d–d, p–α and α–α collisions from 1976 onwards. For CERN’s antiproton programme, a new beamline was built from the PS to Ring 2 for antiproton injection and the first p-p runs took place in 1981. During the ISR’s final year, 1984, the machine was dedicated to single-ring operation with a 3.5 GeV/c antiproton beam.
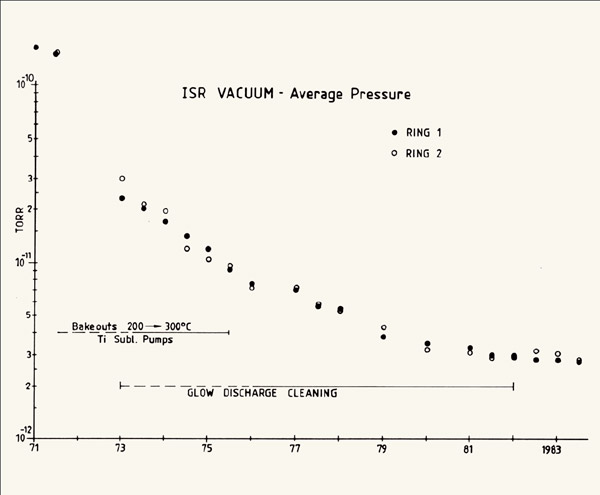
The low loss-rates observed for the gradually rising operational currents were only achievable through a continuous upgrading of the ultra-high vacuum system, which led to a steady decrease in the average pressure (figure 4). The design values for the vacuum pressure were 10–9 torr outside the intersection regions and 10–11 torr in these regions to keep the background under control. The initial choice of a stainless-steel vacuum chamber bakeable to 300°C turned out to be the right one but nevertheless a painstaking and lengthy programme of vacuum improvement had to be launched. The vacuum chambers were initially baked to only 200°C and had to be re-baked at 300°C and, later, at 350°C. Hundreds of titanium sublimation pumps needed to be added and all vacuum components had to be glow-discharge cleaned in a staged programme. These measures limited the amount of residual gas, and hence the production of ions from beam–gas collisions, as well as the out-gassing that occurred when positive ions impinged on the vacuum chamber walls after acceleration through the electrostatic beam potential.
The electrons created by ionization of the residual gas were often trapped in the potential well of the coasting proton beam. This produced an undesirable focusing and coupling between the electron cloud and the beam, which led to “e–p” oscillations. The effect was countered by mounting clearing electrodes in the vacuum chambers and applying a DC voltage to suppress potential wells in the beam.
By 1973 the ISR had suffered two catastrophic events in which the beam burnt holes in the vacuum chamber
By 1973 the ISR had suffered two catastrophic events in which the beam burnt holes in the vacuum chamber. Collimation rings were then inserted into the flanges to protect the bellows. The vacuum and engineering groups also designed and produced large, thin-walled vacuum chambers for the intersection regions. The occasional collapse of such a chamber would leave a spectacular twisted sculpture and require weeks of work to clean the contaminated arcs.
While the ISR broke new ground in many ways, the most important discovery in the field of accelerator physics was that of Schottky noise in the beams – a statistical signal generated by the finite number of particles, which is well known to designers of electronic tubes. This shot noise not only has a longitudinal component but also a transverse component in the betatron oscillations (the natural transverse oscillations of the beam). This discovery opened new vistas for non-invasive beam diagnostics and active systems for reducing the size and momentum-spread of a beam.
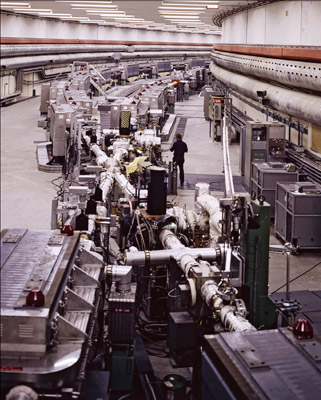
The longitudinal Schottky signal made it possible to measure the current density in the stack as a function of the momentum (transverse position) without perturbing it. These scans clearly showed the beam edges and any markers (figure 5). A marker could be created during stacking by making a narrow region of low current-density or by losses on resonances.
The transverse Schottky signals gave information about how the density of the stack varied with the betatron frequency, or “tune”, which meant that stacking could be monitored in the tune diagram and non-linear resonances could be avoided. During stacking, space–charge forces increase and change the focusing experienced by the beam. Using the Schottky scans as input, the magnet system could be trimmed to compensate the space–charge load. A non-invasive means to verify the effect of space charge and to guide its compensation had suddenly become available.
The discovery of the transverse Schottky signals had another, and arguably more important impact, namely the experimental verification of stochastic cooling of particle beams. This type of cooling was invented in 1972 by Simon van der Meer at CERN. Written up in an internal note, it was first considered as a curiosity without any practical application. However, Wolfgang Schnell realized its vast potential and actively looked for the transverse Schottky signals at the ISR. This was decisive for the resurrection of van der Meer’s idea from near oblivion and its experimental proof at the ISR (figure 6). Towards the end of ISR’s life, stochastic cooling was routinely used on antiproton beams to increase the luminosity in antiproton–proton collisions by counteracting the gradual blow-up of the antiproton beam through scattering with residual gas as well as resonances.

Stochastic cooling was the decisive factor in the conversion of the SPS to a pp collider and in the discovery there in 1983 of the long-sought W and Z bosons. This led to the awarding of the Nobel Prize in Physics to Carlo Rubbia and van der Meer, the following year. The technique also became the cornerstone for the success of the more powerful Tevatron proton–antiproton collider at Fermilab. In addition, CERN’s low-energy antiproton programmes in the Low Energy Antiproton Ring and the Antiproton Decelerator, as well as similar programmes at GSI in Germany and at Brookhaven in the US, owe their existence to stochastic cooling. The extension to bunched beams and to optical frequencies makes stochastic cooling a basic accelerator technology today.
A lasting impact
With its exceptional performance, the ISR dispelled the fears that colliding beams were impractical and dissolved the reluctance of physicists to accept the concept as viable for physics. In addition to stochastic cooling, the machine pioneered and demonstrated large-scale, ultra-high vacuum systems as well as ultra-stable and reliable power converters, low-noise RF systems, superconducting quadrupoles and diagnostic devices such as a precise DC current transformer and techniques such as vertically sweeping colliding beams through each other to measure luminosity – another of van der Meer’s ideas.
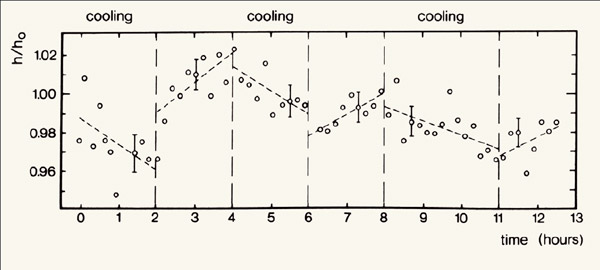
The ISR had been conceived of in 1964 in an atmosphere of growing resentment against the high costs of particle physics and it was in a similar climate in the early 1980s that the rings were closed down to provide financial relief for the new Large Electron–Positron collider at CERN. The political pressures in the 1960s had fought and accepted the ISR as a cost-efficient gap-filler because the financial and political climates were not ready for a 300 GeV machine. However, had CERN built the 300 GeV accelerator instead of the ISR, then the technology of hadron colliders would have been seriously delayed. Instead, the decision to build the ISR opened the door to collider physics and allowed an important expansion in accelerator technology that would affect everyone for the better, including the 300 GeV project, the pp project and eventually the LHC.