The high-luminosity, polarised beams of the proposed International Linear Collider and the triggerless operation of its detectors offer rich physics opportunities beyond its Higgs-factory programme.
The International Linear Collider (ILC) is a proposed electron–positron linear collider with a Higgs factory operating at a centre-of-mass energy of 250 GeV (ILC250) as a first stage. Its electron and positron beams can be longitudinally polarised, and the accelerator may be extended to operate at 500 GeV up to 1 TeV, and possibly beyond. In addition, the unique time structure of the ILC beams (which would collide at short bursts of 1312 bunches with 0.554 ms spacing at a frequency of 5 Hz) places much less stringent requirements on readout speed and radiation hardness than conditions at the LHC detectors. This allows the use of low-mass tracking and high-granularity sensors in the ILC detectors, giving unprecedented resolution in jet-energy measurements. It also results in an expected data rate of just a few GB/s, allowing collisions to be recorded without a trigger.

ILC250 primarily targets precision measurements of the Higgs boson (see Targeting a Higgs factory). However, fully exploiting these measurements demands substantial improvement in our knowledge about many other Standard Model (SM) observables. Here, ILC250 opens three avenues: the study of gauge-boson pair-production and fermion pair-production at 250 GeV; fermion-pair production at effective centre-of-mass energies lowered to about 91.2 GeV by prior emission of photons (radiative returns to the Z pole); and operation of the collider at both the Z pole and the WW threshold. In all of these cases, the polarisation of the electron and positron beams (at polarisations up to 80% and 30%–60%, respectively) boosts the statistical power of many measurements by factors between 2.5 (for Higgs measurements) and 10 (at the Z pole), thanks to the ability to exploit observables such as left–right asymmetries of production cross-sections. These additional polarisation-dependent observables are also essential to disentangle the unavoidable interference between Z and γ exchange in fermion pair-production at energies above the Z pole, enabling access to the chiral couplings of fermions to the Z and the photon. Broadly speaking, the polarised beams and the high luminosity of ILC250 will lead to at least one order of magnitude improvement over the current knowledge for many SM precision observables.
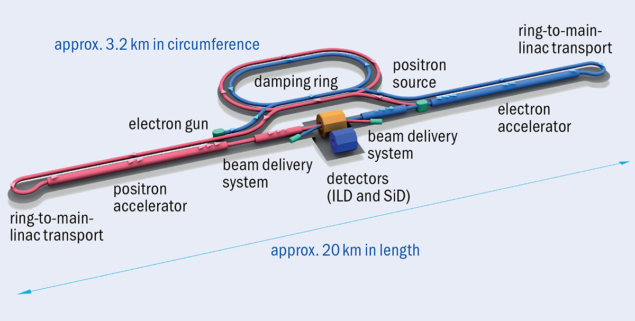
Other important inputs when interpreting Higgs measurements are charged triple-gauge couplings (TGCs), which are also probes of physics beyond the SM. ILC250 will measure these 100 times more precisely than LEP, with a further factor-of-two improvement possible at the higher-energy stage ILC500. These numbers refer to the case of extracting simultaneously all three TGCs relevant in SM effective field theory, which is currently the most favoured framework for the interpretation of precision Higgs-boson data, whereas TGC results from the LHC assume that only one of these couplings deviates from its SM value at a time. With both beams polarised and with full control over the orientation of the polarisation vectors, all 28 TGC parameters that exist in the most general case can potentially be determined simultaneously at the ILC.
Z-pole physics
Classic electroweak precision observables refer to the Z pole. ILC250 will produce about 90 million visible Z events via radiative return, which is about five times more than at LEP and 100 times more than SLC. Thanks to the polarised beams, these data will allow a direct measurement of the asymmetry Ae between the left- and right-handed electron’s coupling to the Z boson with 10 times better accuracy than today, and enable the asymmetries Af of the final-state fermions to the Z to be directly extracted. This is quite different from the case of unpolarised beams, where only the product Ae Af can be accessed. Compared to LEP/SLC results, the Z-pole asymmetries can be improved by typically a factor of 20 using only the radiative returns to the Z at ILC250. This would settle beyond doubt the long-standing question of whether the 3σ tension between the weak mixing-angle extractions from SLC and LEP originates from physics beyond the SM. With a few minor modifications, the ILC can also directly operate at the Z pole, improving fermion asymmetries by another factor 6 to 25 with respect to the radiative-return results.
The higher integrated luminosity of the ILC will provide new opportunities to search for physics beyond the SM
At energies above the Z pole, di-fermion production is sensitive to hypothetical, heavy siblings of the Z boson (so-called Z′ bosons) and to four-fermion operators, i.e. contact-interaction-like parametrisations of yet unknown interactions. ILC250 could indirectly discover Z′ particles with masses up to 6 TeV, while ILC1000 could extend the reach to 18 TeV. For contact interactions, depending on the details of the assumed model, compositeness scales of up to 160 TeV can be probed at ILC250, and up to nearly 400 TeV at ILC1000.
Direct searches for new physics
At first glance, it might seem that direct searches at ILC250 offer only a marginal improvement over LEP, which attained a collision energy of 209 GeV. Nevertheless, the higher integrated luminosity of the ILC (about 2000 times higher than LEP’s above the WW threshold), its polarised beams, much-improved detectors, and triggerless readout will provide new opportunities to search for physics beyond the SM. For example, ILC250 will improve on LEP searches for a new scalar particle produced in association with the Z boson by over an order of magnitude. Another example of a rate-limited search at LEP is the supersymmetric partner of the tau lepton, the tau slepton. In the most general case, tau-slepton masses above 26.3 GeV are not excluded, and in this case no improvement from HL-LHC is expected. The ILC, with its highly-granular detectors covering angles down to 6 mrad with respect to the collision axis, has the ability to cover masses up to nearly the kinematic limit of half the collision energy, also in the experimentally most difficult parts of the parameter space.
The absence of discoveries of new high-mass states at the LHC has led to increased interest in fermionic “Z-portal” models, with masses of dark-matter particles below the electroweak scale. A dark photon, for example, could be detected via its mixing with SM photons. In searching for such phenomena, ILC250 could cover the region between the reach of the B-factories, which is limited to below 10 GeV, and the LHC experiments, which start searching in a range above 150 GeV.

The ILC’s Higgs-factory stage will require only about 40% of the tunnel length available at the Kitakami Mountains in northern Japan, which is capable of housing a linear collider at least 50 km long. This is sufficient to reach a centre-of-mass energy of 1 TeV with current technology by extending the linacs and augmenting power and cryogenics. The upgrade to ILC500 is expected to cost approximately 60% of the ILC250 cost, while going to 1 TeV would require an estimated 100% of the ILC250 cost, assuming a modest increase of the accelerating gradient over what has been achieved (CERN Courier November/December 2020 p35). These upgrades offer the opportunity to optimise the exact energies of the post-Higgs-factory stages according to physics needs and technological advances.
ILC at higher energies
ILC500 targets the energy range 500–600 GeV, which would improve the precision on Higgs-boson couplings typically by a factor of two compared to ILC250 and on charged triple-gauge couplings by a factor of three to four. It would also offer optimal sensitivity in three important measurements. The first is the electroweak couplings of the top quark, for which a variety of new-physics models predict deviations for instance in its coupling to the Z (see “Model sensitivity” figure). The second is the Higgs self-coupling λ from double Higgs-strahlung (e+e– → ZHH): while ILC500 could reach a precision of 27% on λ, at 1 TeV a measurement based on vector-boson fusion (VBF) reaches 10%. These numbers assume that λ takes the value predicted by the SM. However, the situation can be quite different if λ is larger, as is typically required by models of baryogenesis, and only the
combination of double Higgs-strahlung and VBF-based measurements can guarantee a precision of at least 10–20% for any value of λ (see “Higgs self-coupling” figure). A third physics target is the top-quark Yukawa coupling, for which a precision of 6.3% is projected at ILC500, 3.2% at 550 GeV and 1.6% at 1 TeV.
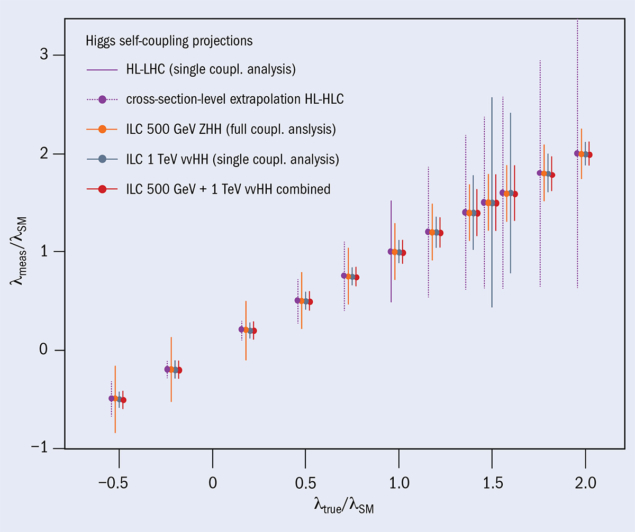
While ILC250 has interesting discovery potential in various rate-limited searches, ILC500 extends the kinematic reach significantly beyond LEP. For instance, in models of supersymmetry that adhere to naturalness, the supersymmetric partners of the Higgs boson (the higgsinos) must have masses that are not too far from the Z or Higgs bosons, typically around 100 to 300 GeV. While the lower range of these particles is already accessible at ILC250, the higher energy stages of the ILC will be able to cover the remainder of this search space. The ILC is also able to reconstruct decay chains when the mass differences among higgsinos are small, which is a challenging signature for the HL-LHC.
The ILC is the only future collider that is currently being discussed at the government level, by Japan, the US and various countries in Europe. It is also the most technologically established proposal, its cutting edge radio-frequency cavities already in operation at the European XFEL. The 2020 update of the European strategy for particle physics also noted that, should an ILC in Japan go ahead, the European particle-physics community would wish to collaborate. Recently, an ILC international development team was established to prepare for the creation of the ILC pre-laboratory, which will make all necessary technical preparations before construction can begin. If intergovernmental negotiations are successful, the ILC could undergo commissioning as early as the mid-2030s.
Further reading
P Bambade et al. 2019 arXiv:1903.01629.
K Fujii et al. 2020 arXiv:2007.03650.
K Fujii et al. 2019 arXiv:1908.11299.