Niobium–copper accelerating cavities, once the pinnacle of radio-frequency technology at CERN, are back in business and beginning to challenge the performance of their bulk-niobium counterparts.
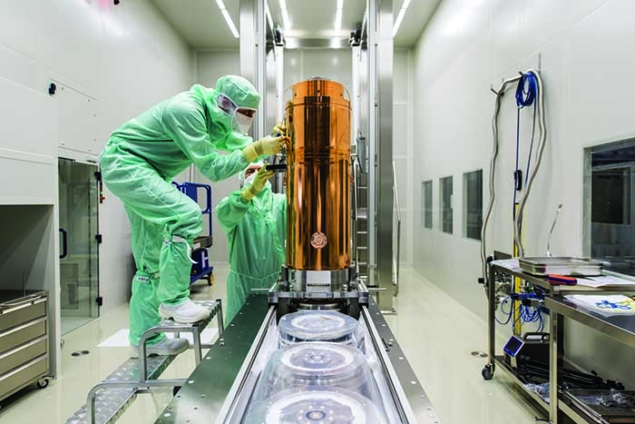
Superconductors are poor thermal conductors. Whenever a superconducting state is established in a given material, a large fraction of the conduction electrons are frozen in Cooper pairs and become unavailable for heat transport. This can have serious practical implications: at low temperatures, even a small amount of localised heating can drive the material into a normal conducting state, triggering an avalanche process (or quench) that destroys superconductivity in the whole device. It is therefore good practice in applied superconductivity to stabilise superconductors with a high-thermal-conductivity metal. The superconducting niobium–titanium filaments in strands used for accelerator magnets such as those in the LHC, for example, are usually embedded in a copper matrix to spread out any small fluctuation in temperature.
In the world of superconducting radio-frequency (SRF) cavities, which are used to accelerate charged particles in accelerators around the globe, this technique is the exception rather than the rule. Today, mainstream SRF technology makes use of bulk niobium sheets to build the entire resonator structure, circumventing the problem of poor thermal conductivity by using material of very high purity. In the past 40 years, great leaps forward have brought bulk-niobium cavity performances close to what is considered the intrinsic limit of the material, with accelerating fields of the order 50 MV/m in elliptical structures.
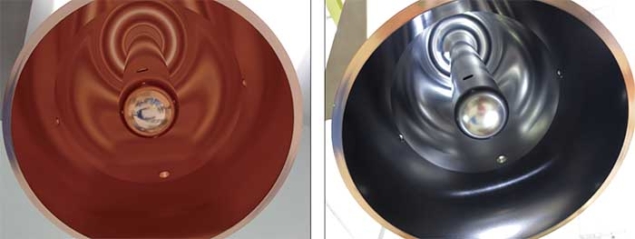
However, things were not always this way. In the 1960s, lead (which is a type-I superconductor) was electroplated on copper RF resonators used for beam acceleration at several high-energy physics facilities around the world. As the RF currents only penetrate a few tens of nanometres in the cavity wall, a few-micron-thin superconducting layer on a high-thermal-conductivity copper substrate provided an elegant solution to the problem of thermal stabilisation, in perfect analogy to what happens in superconducting strands for magnets.
Coated RF cavities (figure 1) offered another advantage: they allowed the function of producing high electric fields to be easily decoupled from that of giving enough mechanical stability, which is required to control the field amplitude and phase sufficiently for beam acceleration. The main drawback of lead-plated cavities was their relatively low accelerating field, limited by the critical magnetic field of lead. A natural step forwards was to use niobium, whose critical field is about 2.5 times higher. Unfortunately, the synthesis of good-quality niobium films on copper was much more difficult, and in the 1970s the research quickly turned to using bulk niobium as a cavity material.
Niobium-coated cavities
In 1984, Cristoforo Benvenuti, Nadia Circelli and Max Hauer from CERN (figure 2) published a seminal paper on niobium films for superconducting accelerating cavities. These films were deposited on copper cavity substrates by sputtering, which was reported to give encouraging results on real cavities. It was the start of a successful development, which in only a few years led to the greatest achievement of niobium–copper technology: the SRF system of the upgraded Large Electron Positron collider (LEP), which operated at CERN in the mid- to late-1990s. This consisted of 288 four-cell elliptical cavities working at a frequency of 352 MHz, the vast majority of which were produced with niobium–copper technology in three European industries. During the early times of LEP, niobium–copper cavities could outperform their bulk niobium counterparts at LEP’s nominal fields. Besides being cheaper and free from quenches, they also revealed unexpected insensitivity to trapped magnetic flux. This is a peculiar problem of superconducting cavities that can spoil their performance unless great care is used to shield the cavity from any magnetic fields when it undergoes the superconducting transition. The need for magnetic shielding added to the cost of the bulk niobium systems, whereas LEP’s niobium–copper cavities could operate without shielding.
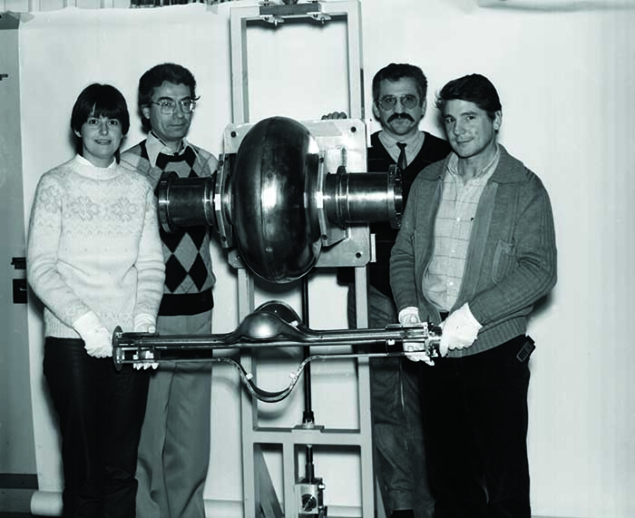
In the meantime, as coated-cavity technology took off at CERN, bulk niobium technology was progressing fast: all over the world in national laboratories and in industry, the SRF community removed all of the obstacles one after the other in the quest for higher accelerating fields and lower power dissipation (expressed by the unloaded quality factor, Q). Nowadays, state-of-the-art bulk niobium cavities are cutting-edge technology objects made from high residual-resistivity-ratio (RRR) niobium sheets that are shaped and electron-beam welded with the utmost precision. They must be assembled according to high cleanliness standards borrowed from the semiconductor industry to prevent electron-field emission. They need to be carefully shielded from the Earth’s and other parasitic magnetic fields, operated in superfluid helium, and employ complex feedback systems and high installed RF power to combat the effects of microphonics (vibrations that detune the cavities). The result is outstanding in terms of accelerating field and Q, now approaching the theoretical limits. Bulk niobium cavities can be produced in industry and are operating reliably in accelerators at several facilities worldwide, such as the European X-ray free electron laser (XFEL) in Hamburg (CERN Courier July/August 2017 p25).

In contrast, niobium–copper cavities suffered from a problem that had been present since the start: at high accelerating fields, the cavity Q value decreased faster than it did in the case of bulk niobium. This phenomenon is still not fully explained today. During and after the LEP era, a great deal of research was carried out at CERN on 1.5 GHz niobium–copper cavities to tackle the issue. Despite significant progress in understanding and remarkable cavity results, the gap in performance compared with bulk niobium was not bridged. This prevented niobium-coated copper cavities from being considered for the high-energy linear colliders under study at the time – namely TESLA, the technology of which has since morphed into that underpinning the European XFEL and the International Linear Collider proposal (CERN Courier September 2017 p27).
At medium accelerating fields, like those required for circular hadron machines and many other applications, the drop in Q was not a showstopper. For the LHC, the niobium–copper technology was applied to build the 16 single-cell 400.8 MHz elliptical cavities required in the machine’s accelerating sections. The LHC cavities, like their LEP ancestors, also work at a temperature of 4.5 K – the cryogenics for which is cheaper and more robust than that used for bulk niobium devices.

In the 1990s, niobium–copper cavities of another shape, adapted for heavy-ion acceleration, were employed for the ALPI linac in INFN Legnaro, Italy. Here, Vincenzo Palmieri and collaborators developed niobium-sputtered quarter-wave resonators (QWRs) on copper substrates. A total of 58 niobium–copper cavities are operational today in ALPI, replacing old lead-plated cavities and considerably extending the energy reach of the machine.
Revival at ISOLDE
During the construction of the LHC in the early 2000s, the SRF activities and infrastructures at CERN were down-sized as resources were focused on the production of the LHC’s superconducting magnets. However, the need for a new SRF system came back in a CERN project in 2009, when a proposal was approved for a high-energy upgrade of the ISOLDE facility using a superconducting linac booster for the radioactive ion beams. For this application, niobium–copper technology was considered particularly well suited because the absence of beam loading allows the stiff, niobium-coated copper cavities to be operated at very narrow RF bandwidths, leading to significant savings in installed RF power. To support the high-energy HIE-ISOLDE upgrade, CERN invested in rebuilding its SRF infrastructure and expertise.
Today, thanks to the collective effort of several CERN teams, the high-beta section of the HIE-ISOLDE linac is complete (figure 3). The work for HIE ISOLDE also offered an opportunity to advance understanding of the limitations to niobium–copper cavity performance. One particular issue was the frequent appearance of defects on the copper substrate, especially close to the electron-beam weld. To overcome this problem, towards the end of the production, a new design for the RF cavity was proposed, which made possible machining the whole resonator out of a copper billet and thus avoided any weld (figure 1). The results of the change were very encouraging.
The first two cavities, manufactured with this technique in industry and coated at CERN, were tested at the end of 2017. Their RF performance scored top of a series of 20 units. Even more strikingly, when cooled down close to superfluid helium temperatures with active shielding of the ambient field, a cavity reached unprecedented peak fields for niobium–copper technology (figure 4). Incredibly, the RF performance of this cavity is comparable to bulk niobium cavities with the same shape, at least as far as the Q-slope is concerned. This result is now the basis of exploring new possible applications, notably for the acceleration of higher-beta beams like those required in spallation sources for accelerator-driven systems.
At about the same time, another excellent result was achieved in the context of the LHC spare-cavities programme. Newly coated cavities are giving results that lie on an upward learning curve, and have already surpassed the LHC specifications. Achieving such good RF performances is only possible if high quality standards are maintained along the whole production chain, from manufacturing of the copper substrate, to chemical polishing, ultra-pure water rinsing, cleanroom assembly and coating, to the final RF test at cryogenic temperatures. This requires a close collaboration of various teams of specialists, and CERN is an ideal place for that.
These two recent achievements are proof that the potential of niobium–copper technology is not exhausted, and that these cavities could be as high performing as their bulk niobium counterparts. Indeed, this technology is already being considered within the Future Circular Collider study, led by CERN to explore the feasibility of a 100 km-circumference machine. Clearly, the long march of niobium on copper is far from over.
Further reading
V Arbet-Engels et al. 2001 Nucl. Instr. Meth. Phys. Res 463 1.
C Benvenuti et al. 1984 App. Phys. Lett. 45 583.
J M Pierce et al. 1965 Proc. 9th Int. Conf. on Low Temperature Physics (vol. l A) Plenum, New York, p36.
J Tuckmantel 1999 IEEE Trans. Appl. Supercond. 9 270.
V Palmieri et al. 1993 Proc. 6th Workshop on RF Superconductivity CEBAF Newport News, Virginia, US.