Detecting and exploring a stochastic background of gravitational waves predicted to pervade the universe potentially opens a window on the extreme physics of the very early universe. Azadeh Maleknejad and Fabrizio Rompineve explain how a new generation of instruments could bring this cosmic symphony into view.

The existence of dark matter in the universe is one of the most important puzzles in fundamental physics. It is inferred solely by means of its gravitational effects, such as on stellar motions in galaxies or on the expansion history of the universe. Meanwhile, non-gravitational interactions between dark matter and the known particles described by the Standard Model have not been detected, despite strenuous and advanced experimental efforts.
Such a situation suggests that new particles and fields, possibly similar to those of the Standard Model, may have been similarly present across the entire cosmological history of our universe, but with only very tiny interactions with visible matter. This intriguing idea is often referred to as the paradigm of dark sectors and is made even more compelling by the lack of new particles seen at the LHC and laboratory experiments so far.
Dark universe
Cosmological observations, above all those of the cosmic microwave background (CMB), currently represent the main tool to test such a paradigm. The primary example is that of dark radiation, i.e. putative new dark particles that, unlike dark matter, behave as relativistic species at the energy scales probed by the CMB. The most recent data collected by the Planck satellite constrain such dark particles to make at most around 30% of the energy of a single neutrino species at the recombination epoch (when atoms formed and the universe became transparent, around 380,000 years after the Big Bang).
While such observations represent a significant advance, the early universe was characterised by temperatures in the MeV range and above (enabling nucleosynthesis), possibly as large as 1016 GeV. Some of these temperatures correspond to energy scales that cannot be probed via the CMB, nor directly with current or prospective particle colliders. Even if new particles had significant interactions with SM particles at such high temperatures, any electromagnetic radiation in the hot universe was continuously scattered off matter (electrons), making it impossible for any light from such early epochs to reach our detectors today. The question then arises: is there another channel to probe the existence of dark sectors in the early universe?
We are entering a golden era of GW observations across the frequency spectrum
For more than a century, a different signature of gravitational interactions has been known to be possible: waves, analogous to those of the electromagnetic field, carrying fluctuations of gravitational fields. The experimental effort to detect gravitational waves (GWs) had a first amazing success in 2015, when waves generated by the merger of two black holes were first detected by the LIGO and Virgo interferometers in the US and Italy.
Now, the GW community is on the cusp of another incredible milestone: the detection of a GW background, generated by all sources of GWs across the history of our universe. Recently, based on more than a decade of observations, several networks of radio telescopes called pulsar timing arrays (PTAs) – NANOGrav in North America, EPTA in Europe, PPTA in Australia and CPTA in China – produced tentative evidence for such a stochastic GW background based on the influence of GWs on pulsars (see “Hints of low-frequency gravitational waves found” and “Clocking gravity” image). Together with next-generation interferometer-based GW detectors such as LISA and the Einstein Telescope, and new theoretical ideas from particle physics, the observations suggest that we are entering an exciting new era of observational cosmology that connects the smallest and largest scales.
Particle physics and the GW background
Once produced, GWs interact only very weakly with any other component of the universe, even at the high temperatures present at the earliest times. Therefore, whereas photons can tell us about the state of the universe at recombination, the GW background is potentially a direct probe of high-energy processes in the very early universe. Unlike GWs that reach Earth from the locations of binary systems of compact objects, the GW background is expected to be mostly isotropic in the sky, very much like the CMB. Furthermore, rather than being a transient signal, it should persist in the sensitivity bands of GW detectors, similar to a noise component but with peculiarities that are expected to make a detection possible.
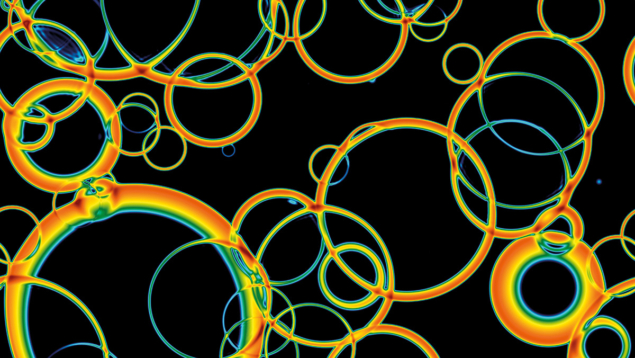
As early as 1918, Einstein quantified the power emitted in GWs by a generic source. Compared to electromagnetic radiation, which is sourced by the dipole moment of a charge distribution, the power emitted in GWs is proportional to the third time derivative of the quadrupole moment of the mass-energy distribution of the source. Therefore, the two essential conditions for a source to emit GWs are that it should be sufficiently far from spherical symmetry and that its distribution should change sufficiently quickly with time.
What possible particle-physics sources would satisfy these conditions? One of the most thoroughly studied phenomena as a source of GWs is the occurrence of a phase transition, typically associated with the breaking of a fundamental symmetry. Specifically, only those phase transitions that proceed via the nucleation, expansion and collision of cosmic bubbles (analogous to the phase transition of liquid water to vapour) can generate a significant amount of GWs (see “Ringing out” image). Inside any such bubble the universe is already in the broken-symmetry phase, whereas beyond the bubble walls the symmetry is still unbroken. Eventually, the state of lowest energy inside the bubbles prevails via their rapid expansion and collisions, which fill up the universe. Even though such bubbles may initially be highly spherical, once they collide the energy distribution is far from being so, while their rapid expansion provides a time variation.
The occurrence of two phase transitions is in fact predicted by the Standard Model (SM): one related to the spontaneous breaking of the electroweak SU(2) × U(1) symmetry, the other associated with colour confinement and thus the formation of hadronic states. However, dedicated analytical and numerical studies in the 1990s and 2000s concluded that the SM phase transitions are not expected to be of first order in the early universe. Rather, they are expected to proceed smoothly, without any violent release of energy to source GWs.
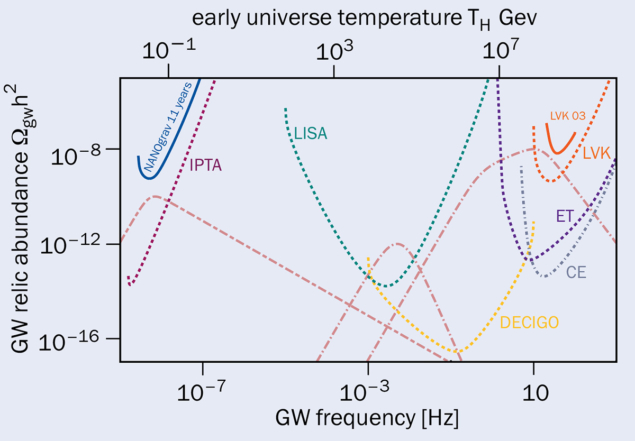
This leads to a striking conclusion: a detection of the GW background would provide evidence for physics beyond the SM – that is, if its origin can be attributed to processes occurring in the early universe. This caveat is crucial, since astrophysical processes in the late universe also contribute to a stochastic GW background.
In order to claim a particle-physics interpretation for any stochastic GW background, it is thus necessary to appropriately account for astrophysical sources and characterise the expected (spectral) shape of the GW signal from early-universe sources of interest. These tasks are being undertaken by a diverse community of cosmologists, particle physicists and astrophysicists at research institutions all around the world, including in the cosmology group in the CERN TH department.
Precise probing
For particle physicists and cosmologists, it is customary to express the strength of a given stochastic GW signal in terms of the fraction of the energy (density) of the universe today carried by those GWs. The CMB already constraints this “relic abundance” to be less than roughly 10% of ordinary radiation, or about one millionth of that of the dominant component of the universe today, dark energy. Remarkably, current GW detectors are already able to probe stochastic GWs that produce only one billionth of the energy density of the universe.
Generally, the stochastic GW signal from a given source extends over a broad frequency range. The spectrum from many early-universe sources typically peaks at a frequency linked to the expansion rate at the time the source was active, redshifted to today. Under standard assumptions, the early universe was dominated by radiation and the peak frequency of the GW signal increases linearly with the temperature. For instance, the GW frequency range in which LIGO/Virgo/KAGRA are most sensitive (10–100 Hz) corresponds to sources that were active when the universe was as hot as 108 GeV – six orders of magnitude higher than the LHC. The other currently operating GW observatories, PTAs, are sensitive to GWs of much smaller frequencies, around 10–9–10–7 Hz, which correspond to temperatures around 10 MeV to 1 GeV (see “Broadband” figure). These are the temperatures at which the QCD phase transition occurred. While, as mentioned above, a signal from the latter is not expected, dark sectors may be active at those temperatures and source a GW signal. In the near (and long-term) future, it is conceivable that new GW observatories will allow us to probe the stochastic GW background across the entire range of frequencies from nHz to 100 Hz.
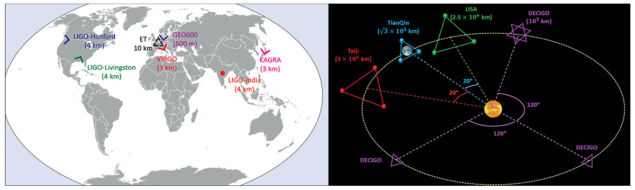
Together with bubble collisions, another source of peaked GW spectra due to symmetry breaking in the early universe is the annihilation of topological defects, such as domain walls separating different regions of the universe (in this case the corresponding symmetry is a discrete symmetry). Violent (so-called resonant) decays of new particles, such as is predicted by some early-universe scenarios, may also strongly contribute to the GW background (albeit possibly only at very large frequencies, beyond the sensitivity reach of current and forecasted detectors). Yet another discoverable phenomenon is the collapse of large energy (density) fluctuations in the early universe, such as is predicted to occur in scenarios where the dark matter is made of primordial black holes.
On the other hand, particle-physics sources can also be characterised by very broad GW spectra without large peaks. The most important such source is the inflationary mechanism: during this putative phase of exponential expansion of the universe, GWs would be produced from quantum fluctuations of space–time, stretched by inflation and continuously re-entering the Hubble horizon (i.e. the causally connected part of the universe at any given time) throughout the cosmological evolution. The amount of such primordial GWs is expected to be small. Nonetheless, a broad class of inflationary models predicts GWs with frequencies and amplitudes such that they can be discovered by future measurements of the CMB. In fact, it is precisely via these measurements that Planck and BICEP/Keck Array have been able to strongly constrain the simplest models of inflation. The GWs that can be discovered via the CMB would have very small frequencies (around 10–17 Hz, corresponding to ~eV temperatures). The full spectrum would nonetheless extend to large frequencies, only with such a small amplitude that detection by GW observatories would be unfeasible (except perhaps for the futuristic Big Bang Observer – a proposed successor to the Laser Interferometer Space Antenna, LISA, currently being prepared by the European Space Agency).
Feeling blue
Certain classes of inflationary models could also lead to “blue-tilted” (i.e. rising with frequency) spectra, which may then be observable at GW observatories. For instance, this can occur in models where the inflaton is a so-called axion field (a generalisation of the predicted Peccei–Quinn axion in QCD). Such scenarios naturally produce gauge fields during inflation, which can themselves act as sources of GWs, with possible peculiar properties such as circular polarisation and non-gaussianities. A final phenomenon that would generate a very broad GW spectrum, unrelated to inflation, is the existence of cosmic strings. These one-dimensional defects can originate, for instance, from the breaking of a global (or gauge) rotation symmetry and persist through cosmological history, analogous to cracks that appear in an ice crystal after a phase transition from water.
Astrophysical contributions to the stochastic GW background are certainly expected from binary black-hole systems. At the frequencies relevant for LIGO/Virgo/KAGRA, such background would be due to black holes with masses of tens of solar masses, whereas in the PTA sensitivity range the background is sourced by binaries of supermassive black holes (with masses up to millions of solar masses), such as those that are believed to exist at the centres of galaxies. The current PTA indications of a stochastic GW background require detailed analyses to understand whether the signal is due to a particle physics or an astrophysics source. A smoking gun for the latter origin would be the observation of significant anisotropies in the signal, as it would come from regions where more binary black holes are clustered.
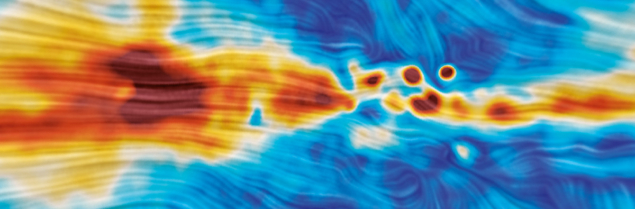
We are entering a golden era of GW observations across the frequency spectrum, and thus in exploring particle physics beyond the reach of colliders and astrophysical phenomena at unprecedented energies. The first direct detection of GWs by LIGO in September 2015 was one of the greatest scientific achievements of the 21st century. The first generation of laser interferometric detectors (GEO600, LIGO, Virgo and TAMA) did not detect any signal and only constrained the gravitational-wave emission from several sources. The second generation (Advanced LIGO and Advanced Virgo) made the first direct detection and has observed almost 100 GW signals to date. The underground Kamioka Gravitational Wave Detector (KAGRA) in Japan joined the LIGO–VIRGO observations in 2020. As of 2021, the LIGO–Virgo–KAGRA collaboration is working to establish the International Gravitational Wave Network, to facilitate coordination among ground-based GW observatories across the globe. In the near future, LIGO India (IndIGO) will also join the network of terrestrial detectors.
Despite being sensitive to changes in the arm length of the order of 10–18 m, the LIGO, Virgo and KAGRA detectors are not sensitive enough for precise astronomical studies of GW sources. This has motivated the new generation of detectors. The Einstein Telescope (ET) is a proposed design concept for a European third-generation GW detector underground, which will be 10 times more sensitive than the current advanced instruments (see “Joined-up thinking in vacuum science”). On Earth, however, gravitational waves with frequencies lower than 1 Hz are inaccessible due to terrestrial gravity gradient noise and limitations to the size of the device. Space-based detectors, on the other hand, can access frequencies as low as 10–4 Hz. Several space-based GW observatories are proposed that will ultimately form a network of laser interferometers in space. They include LISA (planned to launch around 2035), the Deci-hertz Interferometer Gravitational Wave Observatory (DECIGO) led by the Japan Aerospace Exploration Agency and two Chinese detectors, TianQin and Taiji (see “In synch” figure).
Precision detection of the gravitational-wave spectrum is essential to explore particle physics beyond the reach of particle colliders
A new kid on the block, atom interferometry, offers a complementary approach to laser interferometry for the detection of GWs. Two atom interferometers coherently manipulated by the same light field can be used as a differential phase meter tracking the distance traversed by the light field. Several terrestrial cold-atom experiments are under preparation, such as MIGA, ZAIGA and MAGIS, or being proposed, such as ELGAR and AION. These experiments will provide measurements in the mid-frequency range between 10–2–1 Hz. Moreover, a space-based cold-atom GW detector called the Atomic Experiment for Dark Matter and Gravity Exploration (AEDGE) is expected to probe GWs in a much broader frequency range (10–7–10 Hz) compared to LISA.
Astrometry provides yet another powerful way to explore GWs that is not accessible to other probes, i.e. ultra-low frequencies of 10 nHz or less. Here, the passage of a GW over the Earth-star system induces a deflection in the apparent position of a star, which makes it possible to turn astrometric data into a nHz GW observatory. Finally, CMB missions have a key role to play in searching for possible imprints on the polarisation of CMB photons caused by a stochastic background of primordial GWs (see “Acoustic imprints” image). The wavelength of such primordial GWs can be as large as the size of our horizon today, associated with frequencies as low as 10–17 Hz. Whereas current CMB missions allow upper bounds on GWs, future missions such as the ground-based CMB-S4 (CERN Courier March/April 2022 p34) and space-based LiteBIRD observatories will improve this measurement to either detect primordial GWs or place yet stronger upper bounds on their existence.
Outlook
Precision detection of the gravitational-wave spectrum is essential to explore particle physics beyond the reach of particle colliders, as well as for understanding astrophysical phenomena in extreme regimes. Several projects are planned and proposed to detect GWs across more than 20 decades of frequency. Such a wealth of data will provide a great opportunity to explore the universe in new ways during the next decades and open a wide window on possible physics beyond the SM.
Further reading
I Alonso et al. 2022 EPJ Quantum Technol. 9 30.
EPT Collab. 2023 arXiv:2306.16214.
E Komatsu 2022 Nat. Rev. Phys. 4 452.
LISA Collab. 2022 Living Rev. Rel. 25 4.
LiteBIRD Collab. 2023 PTEP 4 042F01.
NANOGrav Collab. 2023 ApJL 951 L8.