Researchers at the Pierre Auger Observatory have taken investigations of air showers created by very-high-energy cosmic rays to new heights. Alan Watson reports on progress.

In 1938, Pierre Auger and colleagues in Paris discovered that showers of cosmic rays can extend over wide areas when they recorded simultaneous events in detectors placed about 30 m apart. Nearly 70 years later, on the pampas of western Argentina, a cosmic-ray observatory bearing Auger’s name is studying extensive air showers over a much wider area, many times the size of Paris itself. These showers are generated by particles with far higher energies than any man-made accelerator can reach, and they continue to challenge our understanding.
The nature and origin of the highest-energy cosmic rays remain obscure. Above 1019 eV (10 EeV) the rate of particles falling on the Earth’s atmosphere is about 1/km2 a year while at 100 EeV, where a small number of particles may have been identified, it falls to less than 1/km2 a century. Thus detectors must be deployed over vast areas to accumulate useful numbers of events. Remarkably, this approach is practical because the cosmic rays generate giant cascades, or air showers, with more than 1010 particles at shower maximum for a 10 EeV primary cosmic ray. Some of the shower particles reach ground-level where they are spread over about 20 km2. The particles also produce fluorescence light by the excitation of atmospheric nitrogen, which provides an alternative and powerful means of detecting the showers and useful complementary information.
The strategy behind the design of the Pierre Auger Observatory is to study showers through detecting not only the particles, with an array of 1600 water Cherenkov detectors, but also the fluorescence light, using four stations, each with six telescopes overlooking the particle detectors. The water tanks are used to measure the energy flow of electrons, photons and muons in the air showers, while the faint light emitted isotropically as the shower moves through the atmosphere can be detected with the fluorescence telescopes. The observatory is now around 70% complete and has been taking data for more than two years.
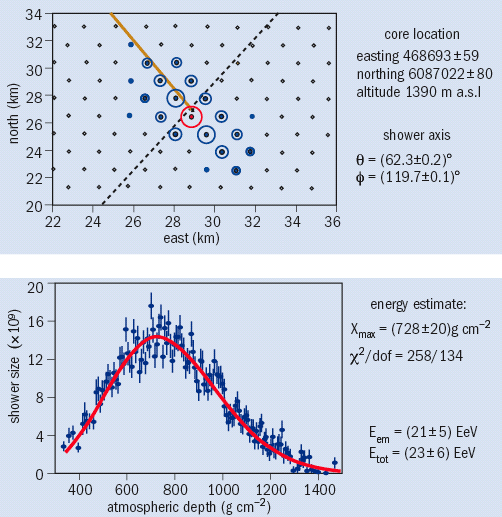
Unlike previous observatories for ultra-high-energy cosmic rays, the Pierre Auger Observatory combines the potential of high statistics from the water tanks, which are on nearly all of the time, with the power of a calorimetric energy determination from the fluorescence devices; it has become known as a hybrid detector. Figure 2. shows an event in which signals are seen in water tanks and in a fluorescence detector.
Alone, the signals from the tanks can be related to the energy of the primary cosmic ray by making assumptions about hadronic interactions. Our limited knowledge about such interactions at the relevant energies will be enhanced by the LHC at CERN, particularly from the forward-physics projects that are being prepared. For now, the energy transferred into the leading particle, the multiplicity and the cross-section for the interaction must all be estimated, while the sparse information on pion-nucleus collisions can be boosted by fixed-target experiments. Additionally, assumptions about the mass of the primary particle must be made, as an iron nucleus, for example, will yield a smaller number of particles at ground level than a proton. With the fluorescence technique, however, these problems can be finessed, and the primary energy can be deduced rather directly.
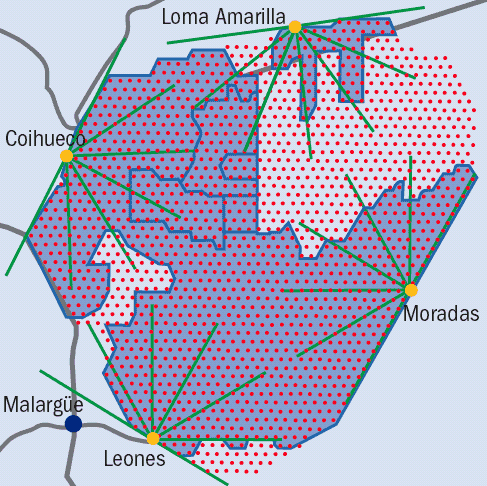
Figure 3 shows the layout of the Pierre Auger Observatory on 31 March, by which time 1113 tanks had been deployed with all but five of them filled with 12 tonnes of pure water; 953 are fitted with electronics and are fully operational. Three of the four fluorescence stations are taking data; the building for the fourth is under construction and telescopes will be installed there in late 2006. When completed, the area covered will be about 30 times bigger than Paris.
All the water tanks operate in an autonomous mode: three 9 inch photomultipliers view each volume of water (10 m2 × 1.2 m) with a trigger rate set at about 20 Hz. The tank signals are calibrated in units of vertical equivalent muons, each of which gives a summed signal of around 300 photoelectrons. The time of each trigger, determined using a GPS receiver, is sent to a central computer using a purpose-built radio system coupled to a microwave link. The computer is used to find detectors clustered in space and time in the manner expected for an air shower, and when a grouping is identified a signal is sent to each detector requesting that other data be transmitted. Currently about 50 events are recorded every hour above a threshold below 1 EeV, with about two events a day from primaries with energies above 10 EeV. Solar cells provide 10 W for the electronics of each tank. Once in position and operational, as in the example in figure 1, these detectors need little attention except replacing the batteries every four years.

A single fluorescence station contains six telescopes, each fitted with a camera that collects light falling on an 11 m2 mirror (see figure 4). The camera has 440 hexagonal photomultipliers (40 mm across), each viewing a different part of the sky. Nitrogen fluorescence at wavelengths of 300-400 nm is observed through a filter, which also keeps out dust. Schmidt optics eliminate coma aberration, achieving a spot size of 0.5°. The trigger for these detectors requires that one of a pre-defined set of patterns is recognized within a group of five photomultiplier pixels. Trigger details are then transmitted to the computer that records the water-tank information. Data from the fluorescence signal about the plane in space that contains the shower direction are combined with the time at which water tanks are struck to define the core position and direction with high precision. The core can be located to about 60 m while the direction is obtained to within around 0.5°. This accuracy is much higher than is possible with either detector type alone. Large showers are sometimes seen in stereo by two fluorescence detectors and a few tri-ocular triggers have been obtained: such events allow the accuracy of event reconstruction to be cross-checked.
A major goal of the Pierre Auger Observatory is to make a reliable measurement of the cosmic-ray energy spectrum above 10 EeV. In particular, the researchers aim to answer the question as to whether or not the spectrum steepens above around 50 EeV, as predicted by Kenneth Greisen, Georgi Zatsepin and Vadim Kuzmin shortly after the discovery of the 2.7 K microwave background. The point is that for protons above this energy the microwave radiation is seen Doppler-shifted to the extent that the Δ+ resonance is excited. This reaction drains a proton of energy so rapidly that for a proton to be detected at 100 EeV, the cosmic-ray source is expected to lie within 50 Mpc. If there are heavy nuclei in the primary beam, they will be fragmented through photo-disintegration, with the diffuse infrared photon field as important as the 2.7 K radiation. The spectrum at the sources will also, of course, be reflected in the details of the spectrum shape.
A further key quantity at the highest energies is the mass of the primary cosmic-ray particles
To determine the spectrum, the Auger Collaboration aim to collect as many events as possible with the surface detectors and to measure the energy of a sub-sample using the fluorescence detectors. The hybrid event shown in figure 2 serves as an example, though in this case reconstruction of the fluorescence light curve, and hence the shape of the cascade, was somewhat simplified because the shower axis was nearly at right-angles to the direction of view. At other orientations the received light is a mixture of fluorescence and Cherenkov radiation arising from high-energy electrons traversing the air. The latter is a particularly serious problem when the trajectory of the shower is towards a telescope.
The data reported so far are from an exposure of 1750 km2 steradian years a year, slightly larger than that achieved by the Akeno Giant Air Shower Array (AGASA) group in Japan. The energy spectrum has been derived from around 3500 events above 3 EeV. Above this energy, the full geometrical area of the detector, defined by the layout of the water tanks, is sensitive so that determination of the flux of events is relatively straightforward. The calibration of the tank signals against the fluorescence detector currently contains relatively large systematic uncertainties (about 30% at 3 EeV and around 50% near 100 EeV), which arise from statistical limitations and uncertainties in the fluorescence yield. The former issue will improve as more data are analysed, while the absolute value of the fluorescence yield is being measured in accelerator laboratories by a small team from the collaboration. Just as with a calorimeter operating in a particle-physics detector, missing energy must be taken into account: although the estimate of this is model- and mass-dependent, the systematic uncertainty in the correction is understood at the 10% level.

The spectrum from the Auger data is shown in figure 5, where it is compared with those from AGASA, HiRes in the US, and the Yakutsk Extensive Air Shower Array in Russia. The general form is similar but, even allowing for the systematic uncertainties still present, it appears that at the highest energies significantly fewer events are seen than expected from the AGASA analysis. The claim of the HiRes team that the spectrum steepens at the highest energies can neither be confirmed nor denied with the present exposure. One event was recorded in April 2004 for which the fluorescence reconstruction gives an energy greater than 140 EeV, but the particle array was small at that date and the shower core fell outside of the fiducial area. Details of the spectrum will be greatly clarified with the data that have been accumulated since June 2005.
A further key quantity at the highest energies is the mass of the primary cosmic-ray particles. This is a significant challenge because of the uncertainties in our understanding of hadronic interactions. Showers produced by iron nuclei will contain more muons than protons, but the magnitude of the difference is relatively small, muons are expensive to identify and model predictions are uncertain. A practical approach is to study the change of the depth of shower maximum as a function of energy. Again it is necessary to make comparisons with models, but here an additional variable – the magnitude of the fluctuation in the depth of shower maximum – is probably less sensitive to details of different models. The fluctuation in the position of shower maximum is smaller for iron nuclei than for protons. To increase statistics, it is desirable to find a parameter measurable with the surface detectors, so the researchers are exploiting both the fall-off of signal size with distance, and features of the time structure of the tank signals measured with 25 ns flash ADCs.
The collaboration has also developed techniques to search for the photon flux that is expected if the highest-energy cosmic rays arise from the decay of super-heavy relic particles, such as cryptons or wimpzillas, which some theorists speculate were produced in the early universe. On average, showers generated by photons are expected to have maxima deeper in the atmosphere by around 200 g/cm2. However, account has to be taken of the orientation of the photon with respect to the Earth’s magnetic field, as electron-pair production is possible and this elevates the depth of maximum. The Landau-Pomeranchuk-Migdal effect must also be accounted for as it leads to significant fluctuations in the shower maximum. A first study has established a limit of 16% above 10 EeV with only 29 events. This limit is not yet very discriminatory, but the technique has significant potential and the result has been submitted to Astroparticle Physics.

Another goal is to search for anisotropies in arrival directions with detection of point sources being the “Holy Grail”. Claims of significant effects at high energies have never been confirmed by independent work with higher statistics. So far, the analysis of data from the Pierre Auger Observatory repeats that story. A search for anisotropies associated with the galactic centre near 1 EeV claimed by the Adelaide group in a re-analysis of material from the Sydney array and by the AGASA group has failed to provide confirmation. Searches at the highest energies have so far been similarly unrewarding.
The Pierre Auger Collaboration is developing the study of inclined events, and showers with zenith angles above 85° have been seen. This was expected as they had been detected long ago with much smaller arrays, but the richness of the new data is impressive. Figure 6 shows an event at about 88° with 31 detectors, and even the present array is too small to contain it. A preliminary estimate of its energy is around 30 EeV. An understanding of these events will lead to additional aperture for collection of the highest-energy particles and also give additional routes to understanding the mass composition. Further, these events form the background against which a neutrino flux might be detectable. There is an exciting future ahead.