QM2012 focuses on the physics of the quark–gluon plasma.
Warning: Undefined array key "attribution" in /opt/app-root/src/wp-content/themes/iopp/inc/shortcodes.php on line 57
Warning: Undefined array key "attribution" in /opt/app-root/src/wp-content/themes/iopp/inc/shortcodes.php on line 57
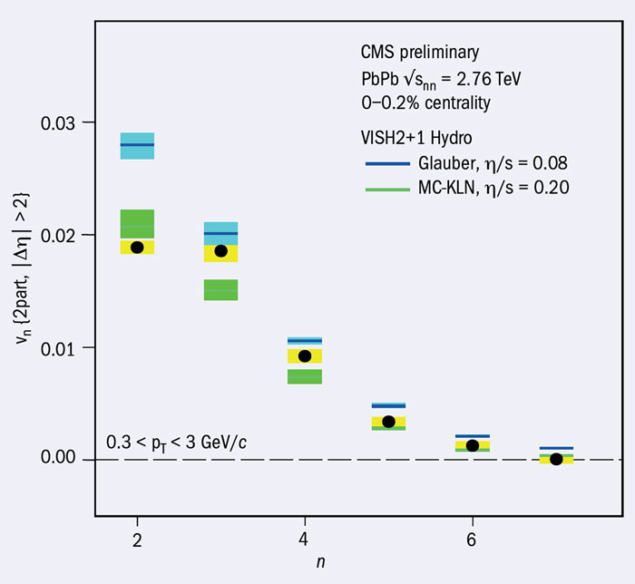
The Quark Matter conferences, held roughly every 18 months, form the most important series of meetings in relativistic heavy-ion physics. The latest and 23rd in the series took place on 13–18 August at the Omni Shoreham hotel, a historic landmark in downtown Washington, DC. The meeting attracted around 700 participants from all around the world who discussed an unprecedented amount of new heavy-ion data from experiments at both Brookhaven National Laboratory (BNL) and CERN. This rich harvest of high-quality experimental results from the PHENIX and STAR collaborations at BNL’s Relativistic Heavy-Ion Collider (RHIC) and the ALICE, ATLAS and CMS collaborations at CERN’s LHC is providing a deep insight into the behaviour of quarks and gluons under the extreme conditions of high temperature and density.
The opening ceremony included presentations by Bart Gordon, former chair of the US House of Representatives Committee on Science and Technology, Timothy Hallman, associate director of Science for Nuclear Physics of the US Department of Energy, and Samuel Aronson, director of BNL. Urs Wiedemann of CERN provided an overview of the current status of relativistic heavy-ion physics, followed by highlights from the experiments presented by Takao Sakaguchi of PHENIX, Xin Dong of STAR, Karel Safarik of ALICE, Barbara Wosiek of ATLAS and Gunther Roland of CMS. The welcome reception was held at the spectacular Smithsonian Institute’s National Portrait Gallery.
Understanding the quark–gluon plasma
Quantum chromodynamics (QCD) – the theory describing the interactions of quarks and gluons – is believed to be responsible for 99% of the mass of the visible universe, with the Higgs boson responsible for the remaining 1%. It has become clear that this mass originates mainly from the self-interaction of gluons, which at short distances is governed by asymptotic freedom. Yet the dynamics of gluon interactions in the large-distance, strong-coupling regime, which is responsible for quark confinement and the existence of atomic nuclei, remains mysterious. It is intimately linked to the complicated and poorly understood structure of the QCD vacuum.
QCD is believed to be responsible for 99% of the mass of the visible universe
The understanding of matter is often advanced by the study of phase transitions in macroscopic systems; thus heavy-ion physics aims towards a better understanding of QCD by creating a “macroscopic” domain of excited vacuum populated by a hot quark–gluon fireball. Advancing the understanding of the quark–gluon plasma also helps in better understanding the origins of the universe – this is because the conditions created in heavy-ion collisions, albeit fleetingly, are similar to the conditions that existed a few microseconds after the Big Bang. In addition, because both QCD and the electroweak sector of the Standard Model are described by non-Abelian gauge theories, understanding the QCD plasma will therefore provide valuable insight into the dynamics of matter at temperatures above the electroweak phase transition that are not accessible in the laboratory. This is important because, for example, the topological “sphaleron” transitions in the electroweak plasma could be responsible for the baryon asymmetry of the present-day universe.

A major advance in the physics of the QCD plasma made possible by the data from RHIC – and now also from the LHC – was the realization that at experimentally accessible temperatures the plasma behaves as a liquid with small dissipation, quantified by small values of shear and bulk viscosities. This implies that there exists a range of temperatures above the deconfinement phase transition in which the plasma does not at all resemble the quasi-ideal gas of quarks and gluons that is expected at high temperatures as a result of asymptotic freedom. At strong coupling, the non-Abelian plasma possesses small shear viscosity as exemplified by the supersymmetric plasma that is amenable to the studies by holographic methods based on string theory. In the latter case, the ratio of shear viscosity to entropy at strong coupling reaches the value of 1/(4π), a value that was conjectured to be the universal lower bound for any fluid. The physics underlying this bound is of a quantum nature because at strong coupling the mean free path approaches the de Broglie wavelength of the constituents, making the quasi-particle picture inapplicable.
The new data presented at Quark Matter 2012 have strengthened the case for the “perfect liquid” and made the physical picture more detailed. The data on hadron spectra and azimuthal correlations from RHIC and the LHC point towards the presence of well localized quantum fluctuations at the early stage of the collision that induce excitations in the quark–gluon liquid. The azimuthal distributions of hadrons are conveniently parameterized by their Fourier coefficients vn. For large n, these coefficients signal the presence of localized fluctuations at the early stage of the collision; their values should be sensitive to the shear viscosity of the liquid.
Ordinarily, the “elliptic flow” v2 dominates over higher harmonics because it reflects decompression of the elliptical shape of the produced fireball. However, all harmonics in the most-central heavy-ion collisions become similar, as illustrated in figure 1 by data from the CMS collaboration in the 0.2% most-central lead–lead (PbPb) collisions at the LHC. A comparison of the data with hydrodynamical calculations shows that the shear viscosity of the liquid is quite close to the conjectured quantum bound, although its precise value depends on the choice of initial conditions.
The initial conditions in heavy-ion collisions are determined by the structure of nuclear wave-functions at small Bjorken-x and the dynamics of their interaction. Significant progress in the understanding of QCD at small x has been made in recent years, triggered by the data from RHIC and the LHC. The quantum evolution in QCD and the high density of partons in Lorentz-contracted nuclei (which can be described as the “colour glass condensate”) lead to the emergence of strong colour fields that dominate the early moments of heavy-ion collisions. The data on the collective flow and other observables suggest that thermalization occurs very early on – within 1 fm/c of the beginning of the collision. The dynamics of this “early thermalization” is not yet entirely understood but several promising theoretical developments were reported at the conference.
One of the proposed signatures of the colour glass condensate is the disappearance of quantum back-to-back di-jet correlations in the forward rapidity region of deuterium–gold collisions at RHIC, owing to the emergence of a semi-classical gluon field at small Bjorken-x. Both the PHENIX and STAR collaborations reported on observations of this effect at RHIC.
The QCD medium can be studied using hard probes to investigate its response to external localized perturbations. The RHIC experiments observed the strong quenching of high-transverse-momentum hadrons and jets that had been proposed as a signature of hot and dense quark–gluon matter. The LHC has significantly extended the kinematic reach in the studies of jets. All LHC experiments found that the strong suppression of jets persists up to high jet energies.
There is no clear sign of the dependence of jet-energy loss on the colour charge of the parton
The mechanism behind the jet-energy loss is still not clear: does it depend on the colour charge of the leading parton (quark or gluon)? Is it suppressed for heavy quark jets, as expected for the medium-induced gluon radiation as a result of the “dead cone” effect? Are the dynamics of energy loss adequately described by perturbative QCD, or does it call for new strong-coupling methods? These questions can be answered only after more detailed data are acquired on jet shapes and flavour-tagged jets.
An interesting effect of the modification of the jet-fragmentation function in PbPb collisions was reported at the conference by the ATLAS and CMS collaborations. Figure 2 shows the ATLAS result. In addition to the enhancement of hadron production at a small fraction of the jet energy z, there is also a sizable dip for the intermediate values of z, which has yet to be understood.
As for the flavour-tagged jets, high-energy b- and c-tagged jets are seen by the LHC experiments to be quenched similarly to the inclusive jets, which are dominated by gluons. At present there is no clear sign of the dependence of jet-energy loss on the colour charge of the parton. At transverse momenta below 8 GeV, there is a hint of weaker quenching for D mesons than for light hadrons, as reported by the ALICE collaboration. The electrons from heavy-flavour decays that receive a significant contribution from beauty decays at high transverse momenta have been found by ALICE to be quenched less than the charm decays of D mesons, as figure 3 shows. This suggests that the quenching of bottom quarks is weaker than that of charm quarks.
The PHENIX collaboration presented the first data on heavy-meson quenching from decay electrons obtained by using their new silicon vertex detector. In accord with the expectations from theory, D mesons are observed to be suppressed less than light hadrons. However, the PHENIX collaboration found surprising hints of a significantly stronger suppression of B-mesons.

An important baseline for jet quenching is provided by the colourless probes – the photons, Z and W bosons. Indeed, the ATLAS and CMS collaborations reported the production of Z bosons with no sign of suppression up to transverse momenta of about 100 GeV. This implies that the observed suppression of jets is, indeed, a result of the colour dynamics.
Heavy quarkonium has been proposed as a probe of deconfinement – the Debye screening in the quark–gluon plasma (QGP) is expected to make quarkonium formation impossible. Strong suppression of J/ψ production was observed at CERN’s Super Proton Synchrotron – and then at RHIC and at the LHC. Studies of heavy quarkonium have now been extended to the bottomonium family, with the expected hierarchy of suppression, as shown in figure 4a from the CMS collaboration: it is more difficult to dissolve states with larger binding energies and smaller radii.
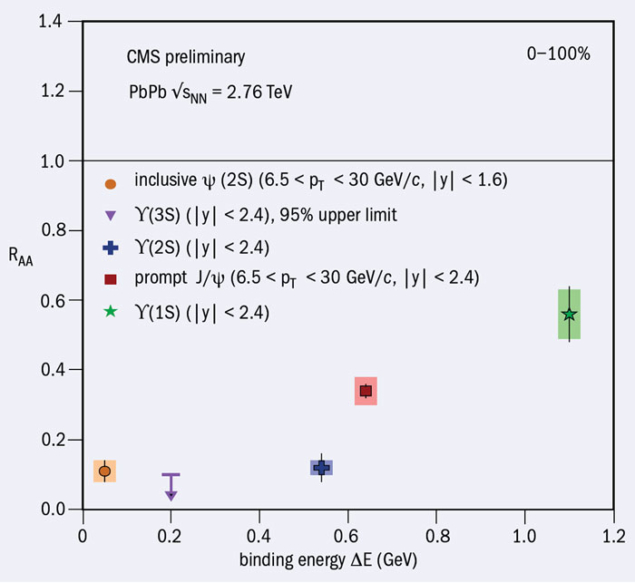
Nevertheless, the observed suppression stems from a complicated interplay of final- and initial-state effects, as suggested by the recent PHENIX data on J/ψ production in asymmetrical copper–gold (CuAu) collisions presented at the conference (figure 4b). The J/ψ suppression at rapidities in the Cu fragmentation region is found to be stronger than in the Au-fragmentation region. This is inconsistent with a final-state effect alone because the density of produced particles is larger in the Au-fragmentation region. On the other hand, J/ψ production in central CuAu collisions in the Cu-fragmentation region selects a high-density region in the wave function at small x of the Au nucleus. The rescattering of heavy quarks in this dense gluon system before the formation of the QGP is expected to reduce the probability for J/ψ formation.
Fluctuations, broken symmetries and the critical point
An important goal of heavy-ion physics is to map the QCD phase diagram. A prominent feature of this phase diagram is the possible existence of a critical point at finite baryon density at the end of the first-order phase-transition curve. The signature of the critical point is the enhancement of fluctuations, including the fluctuations of net baryon number. Experimental access to the high baryon-density in heavy-ion collisions requires decreasing the energy of the collisions at RHIC. The search for the critical point, and thus for the disappearance of signatures of deconfined matter, was the goal of the recent scan of the beam energy at RHIC.
Both the STAR and PHENIX collaborations reported results from the RHIC Beam Energy Scan at the conference. The STAR experiment sees an intriguing deviation of the higher moments of net proton-fluctuations from the Poisson baseline and from the expectations based on Monte-Carlo models at centre-of-mass energies below 20 GeV. A measurement at higher statistics with a finer step in collision energy will be needed to tell whether this observation does point to the existence of the critical point. As the energy of the collision was decreased, several signatures of the plasma phase were found to disappear, as reported by STAR. These include the suppression of the high-transverse momentum hadrons, the scaling in constituent number of the elliptic flow and the fluctuations of charge separation, which is a consequence of the chiral magnetic effect.
Theoreticians have proposed the existence of quantum fluctuations of topological origin in the early stage of heavy-ion collisions, which generate chirality similarly to the electroweak sphalerons that generate baryon number at much higher temperatures. In the presence of the strong magnetic field generated by the colliding heavy ions, the fluctuations in net chirality can lead to fluctuations in the electric-charge separation because of the “chiral magnetic effect”. The resulting observable is the event-by-event fluctuation in the electric-charge separation relative to the reaction plane, signalling the fluctuating electric dipole moment of the plasma. The effect can be accessed experimentally by measuring the difference in the fluctuations of the parity-odd harmonics of azimuthal distributions for hadrons of the same and opposite charge. The effect has been seen at RHIC by the STAR and PHENIX experiments; an effect of similar strength was also reported by the ALICE collaboration.
However, because the observable is parity-even it can receive contributions from more mundane effects. An alternative conventional explanation has been put forward based on the combination of correlations between opposite electric charges and the elliptic flow. Usually, the elliptic flow is correlated with the magnetic field by the geometry of the collision and both vanish in central collisions. However, the new RHIC data on uranium–uranium (UU) collisions allow separation of the two effects. Because of the deformed shape of the uranium nucleus, the central collisions produce a deformed fireball leading to a sizeable elliptic flow; yet, the number of spectators detected by the Zero Degree Calorimeter is small, so the magnetic field must be greatly suppressed. Thus, it should be possible to establish whether the observed fluctuations in charge asymmetries are driven by the elliptic flow or by the magnetic field.
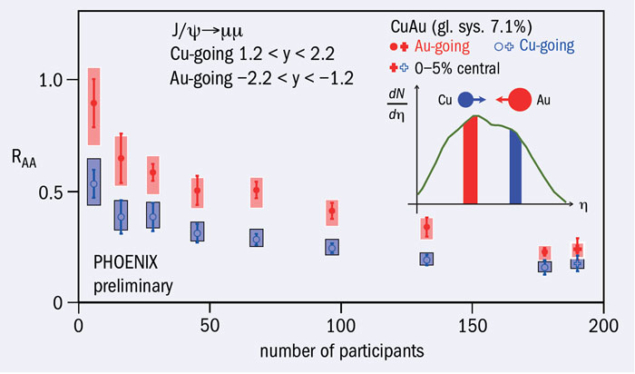
Preliminary data from STAR presented at the conference indicate that the difference in the fluctuations of the asymmetry for the same- and opposite-charge hadrons vanishes in central UU collisions (figure 5), suggesting that these fluctuations are driven by the magnetic field. Another important result on this topic reported by STAR was the difference between the elliptic flows of positive and negative pions in AuAu collisions at 200 GeV, which is found to be linearly dependent on the charge asymmetry in the event, as expected on the basis of the chiral magnetic effect. New refined data are necessary to reach a definitive conclusion on this issue. Topological transitions in QCD generating chirality are analogous to the electroweak sphaleron transitions that generated the baryon asymmetry of the universe shortly after the Big Bang. Therefore, understanding them better is important.
Broad connections
The conference highlighted the broad connections of relativistic heavy-ion physics to condensed-matter physics, string theory, cosmology and astrophysics. For example, the small viscosity of the QGP makes it similar to such seemingly distant objects as ultracold atoms and graphene, where the charge carriers are chiral and the effective coupling is large. The non-dissipative chiral magnetic current appears to exist also in Weyl semimetals and opens possibilities for the creation of a new generation of electronic devices.

The conference made clear the need for dedicated future facilities, several of which were discussed, including: the Electron–Ion Collider needed for a precision study of small-x gluon wave-functions of nuclei and of the spin structure of the proton; the Large Hadron–Electron Collider at CERN, which would advance the high-energy, high-momentum-transfer frontier of deep-inelastic scattering; the Facility for Antiproton and Ion Research under construction at GSI in Darmstadt; and the Nuclotron-based Ion Collider facility, currently under construction in Dubna. The case for the latter two facilities was advanced by the first results from the beam-energy scan at RHIC that were reported at the conference.
Summaries of the results presented were provided by three pairs of rapporteurs, each pair composed of a theorist and experimentalist: Boris Hippolyte of the Institut Pluridisciplinaire Hubert Curien and Dirk Rischke of the University of Frankfurt on global variables and correlations; Jorge Casalderrey-Solana of the University of Barcelona and Alexander Milov of the Weizmann Institute of Science on high-transverse-momenta and jets; and Charles Gale of McGill University and Lijuan Ruan of BNL on heavy flavours, quarkonia and electroweak probes. The wealth of new data and the resulting leap in the theoretical understanding of QCD matter were possible only because of the successes of the two complementary experimental programmes at RHIC and the LHC.