A pileup of 1000 proton–proton collisions per bunch-crossing, highly boosted objects and radiation levels up to 1018 hadrons per cm2 are just some of the challenges in extracting physics from a next-generation hadron collider to follow the LHC. Martin Aleksa, Werner Riegler and Michele Selvaggi digest the conceptual design report for a general-purpose experiment at the Future Circular Collider.
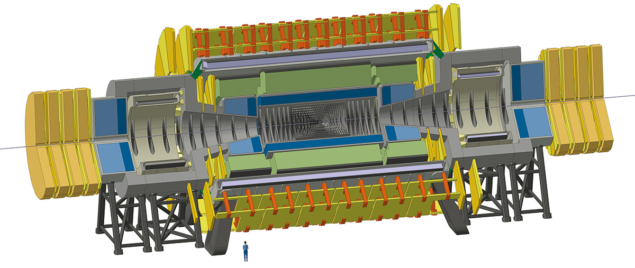
The Future Circular Collider (FCC) is the most powerful post-LHC experimental infrastructure proposed to address key open questions in particle physics. Under study for almost a decade, it envisions an electron–positron collider phase, FCC-ee, followed by a proton–proton collider in the same 91 km-circumference tunnel at CERN. The hadron collider, FCC-hh, would operate at a centre-of-mass energy of 100 TeV, extending the energy frontier by almost an order of magnitude compared to the LHC, and provide an integrated luminosity a factor of 5–10 larger. The mass reach for direct discovery at FCC-hh will reach several tens of TeV and allow, for example, the production of new particles whose existence could be indirectly exposed by precision measurements at FCC-ee.
The potential of FCC-hh offers an unprecedented opportunity to address fundamental unknowns about our universe
At the time of the kickoff meeting for the FCC study in 2014, the physics potential and the requirements for detectors at a 100 TeV collider were already heavily debated. These discussions were eventually channelled into a working group that provided the input to the 2020 update of the European strategy for particle physics and recently concluded with a detailed writeup in a 300-page CERN Yellow Report. To focus the effort, it was decided to study one reference detector that is capable of fully exploiting the FCC-hh physics potential. At first glance it resembles a super CMS detector with two LHCb detectors attached (see “Grand designs” image). A detailed detector performance study followed, allowing a very efficient study of the key physics capabilities.
The first detector challenge at FCC-hh is related to the luminosity, which is expected to reach 3 × 1035 cm–2s–1. This is six times larger than the HL-LHC luminosity and 30 times larger than the nominal LHC luminosity. Because the FCC will operate beams with a 25 ns bunch spacing, the so-called pile-up (the number of pp collisions per bunch crossing) scales by approximately the same factor. This results in almost 1000 simultaneous pp collisions, requiring a highly granular detector. Evidently, the assignment of tracks to their respective vertices in this environment is a formidable task.
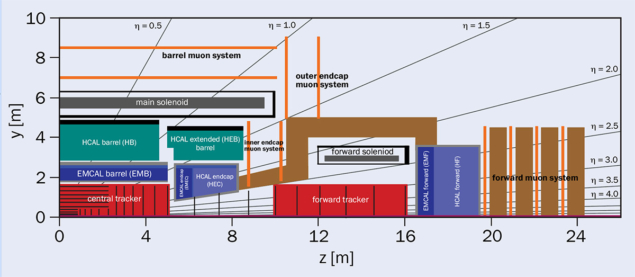
The plan to collect an integrated pp luminosity of 30 ab–1 brings the radiation hardness requirements for the first layers of the tracking detector close to 1018 hadrons/cm2, which is around 100 times more than the requirement for the HL-LHC. Still, the tracker volume with such high radiation load is not excessively large. From a radial distance of around 30 cm outwards, radiation levels are already close to those expected for the HL-LHC, thus the silicon technology for these detector regions is already available.
The high radiation levels also need very radiation-hard calorimetry, making a liquid-argon calorimeter the first choice for the electromagnetic calorimeter and forward regions of the hadron calorimeter. The energy deposit in the very forward regions will be 4 kW per unit of rapidity and it will be an interesting task to keep cryogenic liquids cold in such an environment. Thanks to the large shielding effect of the calorimeters, which have to be quite thick to contain the highest energy particles, the radiation levels in the muon system are not too different from those at the HL-LHC. So the technology needed for this system is available.
Looking forward
At an energy of 100 TeV, important SM particles such as the Higgs boson are abundantly produced in the very forward region. The forward acceptance of FCC-hh detectors therefore has to be much larger than at the LHC detectors. ATLAS and CMS enable momentum measurements up to pseudorapidities (a measure of the angle between the track and beamline) of around η = 2.5, whereas at FCC-hh this will have to be extended to η = 4 (see “Far reaching” figure). Since this is not achievable with a central solenoid alone, a forward magnet system is assumed on either side of the detector. Whether the optimum forward magnets are solenoids or dipoles still has to be studied and will depend on the requirements for momentum resolution in the very forward region. Forward solenoids have been considered that extend the precision of momentum measurements by one additional unit of rapidity.
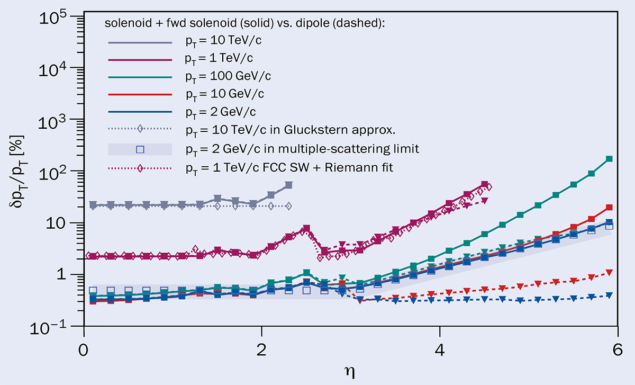
A silicon tracking system with a radius of 1.6 m and a total length of 30 m provides a momentum resolution of around 0.6% for low-momentum particles, 2% at 1 TeV and 20% at 10 TeV (see “Forward momentum” figure). To detect at least 90% of the very forward jets that accompany a Higgs boson in vector-boson-fusion production, the tracker acceptance has to be extended up to η = 6. At the LHC such an acceptance is already achieved up to η = 4. The total tracker surface of around 400 m2 at FCC-hh is “just” a factor two larger than the HL-LHC trackers, and the total number of channels (16.5 billion) is around eight times larger.
It is evident that the FCC-hh reference detector is more challenging than the LHC detectors, but not at all out of reach. The diameter and length are similar to those of the ATLAS detector. The tracker and calorimeters are housed inside a large superconducting solenoid 10 m in diameter, providing a magnetic field of 4 T. For comparison, CMS uses a solenoid with the same field and an inner diameter of 6 m. This difference does not seem large at first sight, but of course the stored energy (13 GJ) is about five times larger than the CMS coil, which needs very careful design of the quench protection system.
For the FCC-hh calorimeters, the major challenge, besides the high radiation dose, is the required energy resolution and particle identification in the high pile-up environment. The key to achieve the required performance is therefore a highly segmented calorimeter. The need for longitudinal segmentation calls for a solution different from the “accordion” geometry employed by ATLAS. Flat lead/steel absorbers that are inclined by 50 degrees with respect to the radial direction are interleaved with liquid-argon gaps and straight electrodes with high-voltage and signal pads (see “Liquid argon” figure). The readout of these pads on the back of the calorimeter is then possible thanks to the use of multi-layer electrodes fabricated as straight printed circuit boards. This idea has already been successfully prototyped within the CERN EP detector R&D programme.
The considerations for a muon system for the reference detector are quite different compared to the LHC experiments. When the detectors for the LHC were originally conceived in the late 1980s, it was not clear whether precise tracking in the vicinity of the collision point was possible in this unprecedented radiation environment. Silicon detectors were excessively expensive and gas detectors were at the limit of applicability. For the LHC detectors, a very large emphasis was therefore put on muon systems with good stand-alone performance, specifically for the ATLAS detector, which is able to provide a robust measurement of, for example, the decay of a Higgs particle into four muons, with the muon system alone.
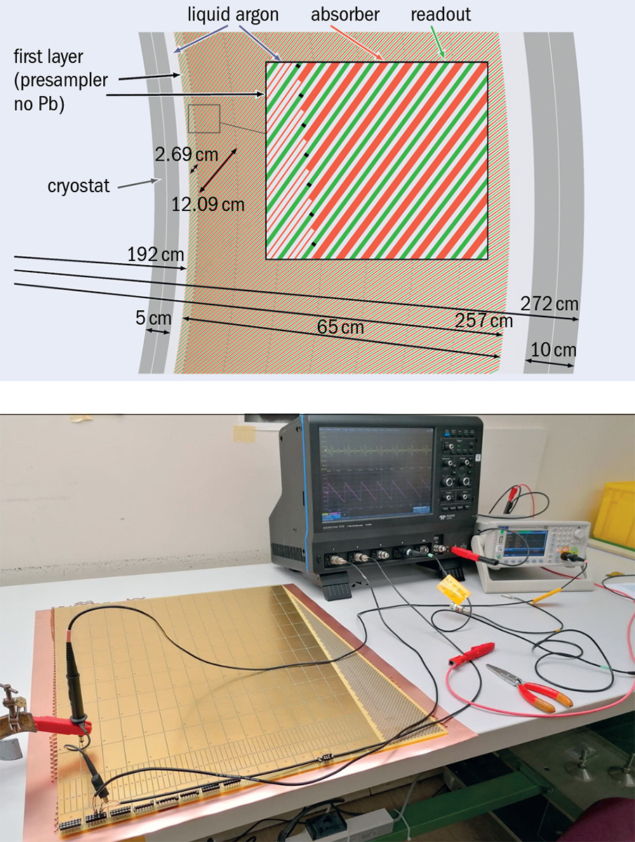
Thanks to the formidable advancement of silicon-sensor technology, which has led to full silicon trackers capable of dealing with around 140 simultaneous pp collisions every 25 ns at the HL-LHC, standalone performance is no longer a stringent requirement. The muon systems for FCC-hh can therefore fully rely on the silicon trackers, assuming just two muon stations outside the coil that measure the exit point and the angle of the muons. The muon track provides muon identification, the muon angle provides a coarse momentum measurement for triggering and the track position provides improved muon momentum measurement when combined with the inner tracker.
The major difference between an FCC-hh detector and CMS is that there is no yoke for the return flux of the solenoid, as the cost would be excessive and its only purpose to shield the magnetic field towards the cavern. The baseline design assumes the cavern infrastructure can be built to be compatible with this stray field. Infrastructure that is sensitive to the magnetic field will be placed in the service cavern 50 m from the solenoid, where the stray field is sufficiently low.
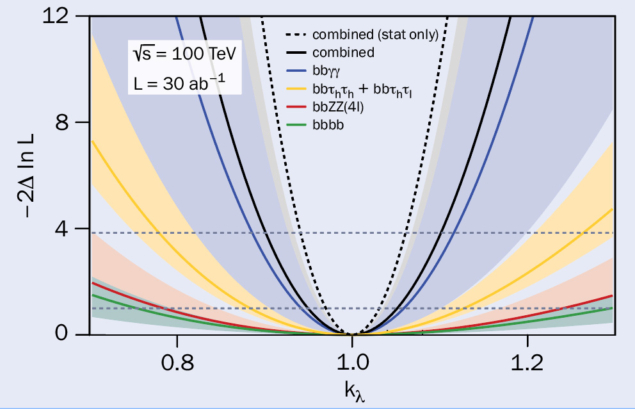
The high granularity and acceptance of the FCC-hh reference detector will result in about 250 TB/s of data for calorimetry and the muon system, about 10 times more than the ATLAS and CMS HL-LHC scenarios. There is no doubt that it will be possible to digitise and read this data volume at the full bunch-crossing rate for these detector systems. The question remains whether the data rate of almost 2500 TB/s from the tracker can also be read out at the full bunch-crossing rate or whether calorimeter, muon and possible coarse tracker information need to be used for a first-level trigger decision, reducing the tracker readout rate to the few MHz level, without the loss of important physics. Even if the optical link technology for full tracker readout were available and affordable, sufficient radiation hardness of devices and infrastructure constraints from power and cooling services are prohibitive with current technology, calling for R&D on low-power radiation-hard optical links.
Benchmarks physics
The potential of FCC-hh in the realms of precision Higgs and electroweak physics, high mass reach and dark-matter searches offers an unprecedented opportunity to address fundamental unknowns about our universe. The performance requirements for the FCC-hh baseline detector have been defined through a set of benchmark physics processes, selected among the key ingredients of the physics programme. The detector’s increased acceptance compared to the LHC detectors, and the higher energy of FCC-hh collisions, will allow physicists to uniquely improve the precision of measurements of Higgs-boson properties for a whole spectrum of production and decay processes complementary to those accessible at the FCC-ee. This includes measurements of rare processes such as Higgs pair-production, which provides a direct measure of the Higgs self-coupling – a crucial parameter for understanding the stability of the vacuum and the nature of the electroweak phase transition in the early universe – with a precision of 3 to 7% (see “Higgs self-coupling” figure).

Moreover, thanks to the extremely large Higgs-production rates, FCC-hh offers the potential to measure rare decay modes in a novel boosted kinematic regime well beyond what is currently studied at the LHC. These include the decay to second-generation fermions, muons, which can be measured to a precision of 1%. The Higgs branching fraction to invisible states can be probed to a value of 10–4, allowing the parameter space for dark matter to be further constrained. The much higher centre-of-mass energy of FCC-hh, meanwhile, significantly extends the mass reach for discovering new particles. The potential for detecting heavy resonances decaying into di-muons and di-electrons extends to 40 TeV, while for coloured resonances like excited quarks the reach extends to 45 TeV, thus extending the current limit by almost an order of magnitude. In the context of supersymmetry, FCC-hh will be capable of probing stop squarks with masses up to 10 TeV, also well beyond the reach of the LHC.
In terms of dark-matter searches, FCC-hh has immense potential – particularly for probing scenarios of weakly interacting massive particles such as higgsinos and winos (see “Dark matters” figure). Electroweak multiplets are typically elusive, especially in hadron collisions, due to their weak interactions and large masses (needed to explain the relic abundance of dark matter in our universe). Their nearly degenerate mass spectrum produces an elusive final state in the form of so-called “disappearing tracks”. Thanks to the dense coverage of the FCC-hh detector tracking system, a general-purpose FCC-hh experiment could detect these particle decays directly, covering the full mass range expected for this type of dark matter.
A detector at a 100 TeV hadron collider is clearly a challenging project. But detailed studies have shown that it should be possible to build a detector that can fully exploit the physics potential of such a machine, provided we invest in the necessary detector R&D. Experience with the Phase-II upgrades of the LHC detectors for the HL-LHC, developments for further exploitation of the LHC and detector R&D for future Higgs factories will be important stepping stones in this endeavour.
Further reading
M Mangano and W Riegler (Eds.) 2022 CERN Yellow Reports: Monographs CERN-2022-002.