Former CERN physicist Christian Fabjan takes a whirlwind tour of 60 years of innovation in particle-detection technology at CERN and beyond.
Particle physics began more than a century ago with the discoveries of radioactivity, the electron and cosmic rays. Photographic plates, gas-filled counters and scintillating substances were the early tools of the trade. Studying cloud formation in moist air led to the invention of the cloud chamber, which, in 1932, enabled the discovery of the positron. The photographic plate soon morphed into nuclear-emulsion stacks, and the Geiger tube of the Geiger–Marsden–Rutherford experiments developed into the workhorse for cosmic-ray studies. The bubble chamber, invented in 1952, represented the culmination of these “imaging detectors”, using film as the recording medium. Meanwhile, in the 1940s, the advent of photomultipliers had opened the way to crystal-based photon and electron energy measurements and Cherenkov detectors. This was the toolbox of the first half of the 20th century, credited with a number of groundbreaking discoveries that earned the toolmakers and their artisans more than 10 Nobel Prizes.
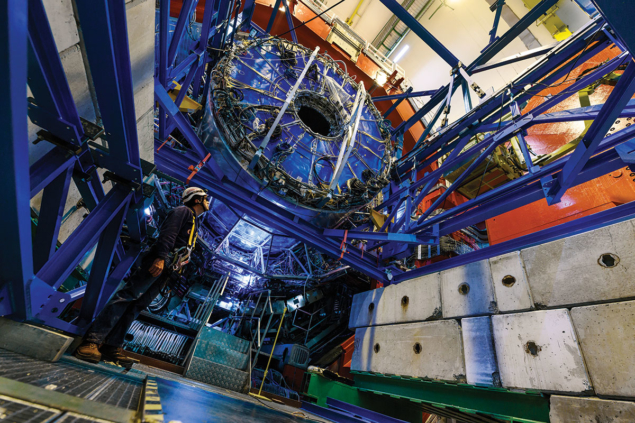
Game changer
The invention of the Multi Wire Proportional Chamber (MWPC) by Georges Charpak in 1968 was a game changer, earning him the 1992 Nobel Prize in Physics. Suddenly, experimenters had access to large-area charged particle detectors with millimetre spatial resolution and staggering MHz-rate capability. Crucially, the emerging integrated-circuit technology could deliver amplifiers so small in size and cost to equip many thousands of proportional wires. This ingenious and deceptively simple detector is relatively easy to construct. The workshops of many university physics departments could master the technology, attracting students and “democratising” particle physics. So compelling was experimentation with MWPCs that within a few years, large detector facilities with tens of thousands of wires were constructed – witness the Split Field Magnet at CERN’s Intersecting Storage Rings (ISR). Its rise to prominence was unstoppable: it became the detector of choice for the Proton Synchrotron, Super Proton Synchrotron (SPS) and ISR programmes. An extension of this technique is the drift chamber, a MWPC-type geometry, with which the time difference between the passage of the particle and the onset of the wire signal is recorded, providing a measure of position with 100 µm-level resolution. The MWPC concept lends itself to a multitude of geometries and has found its “purest” application as the readout of time projection chambers (TPCs). Modern derivatives replace the wire planes with metallised foils with holes in a sub-millimetre pattern, amplifying the ionisation signals.
The ambition, style and success of these large, global collaborations was contagious
The ISR was a hotbed for accelerator and detector inventions. The world’s first proton–proton collider, an audacious project, was clearly ahead of its time and the initial experiments could not fully exploit its discovery potential. It prompted, however, the concept of multi-purpose facilities capable of obtaining “complete” collision information. For the first time, a group developed and used transition-radiation detectors for electron detection and liquid-argon calorimetry. The ISR’s Axial Field Spectrometer (AFS) provided high-quality hadron calorimetry with close to 4π coverage. These technologies are now widely used at accelerators and for non-accelerator experiments. The stringent performance requirements for experiments at the ISR encouraged the detector developers to explore and reach a measurement quality only limited by the laws of detector physics: science-based procedures had replaced the “black magic” of detector construction. With collision rates in the 10 MHz range, these experiments (and the ISR) were forerunners of today’s Large Hadron Collider (LHC) experiments. Of course, the ISR is most famous for its seminal accelerator developments, in particular the invention of stochastic cooling, which was the enabling technology for converting the SPS into a proton–antiproton collider.
The SPS marked another moment of glory for CERN. In 1976 first beams were accelerated to 400 GeV, initiating a diverse physics programme and motivating a host of detector developments. Advances in semiconductor technology led to the silicon-strip detector. With the experiments barely started, Carlo Rubbia and collaborators launched the idea, as ingenious as it was audacious, to convert the SPS into a proton–antiproton collider. The goal was clear: orchestrate quickly and rather cheaply a machine with enough collision energy to produce the putative W and Z bosons. Simon van der Meer’s stochastic-cooling scheme had to deliver the required beam intensity and lifetime, and two experimental teams were charged with the conception and construction of the equally novel detectors. The centrepiece of the UA1 detector was a 6 m-long and 2 m-diameter “electronic bubble chamber”, which adapted the drift-chamber concept to the event topology and collision rate, combined with state-of-the-art electronic readout. The electronic images were of such illuminating quality that “event scanning”, the venerable bubble- chamber technique, was again a key tool in data analysis. The UA2 team pushed calorimetry and silicon detectors to new levels of performance, provided healthy competition and independent discoveries. The discovery of the W and Z bosons was achieved in 1983 and, the following year, Rubbia and van der Meer became Nobel Laureates.
Laying foundations
In 1981, with the approval of the Large Electron Positron (LEP) collider, the community laid the foundation for decades of research at CERN. Mastering the new scale of the accelerator dimension also brought a new approach to managing the larger experimental collaborations and to meeting their more stringent experimental requirements. For the first time, mostly outside collaborators developed and built the experimental apparatus, a non-trivial, but needed success in technology transfer. The detection techniques reached a new state of matureness. Silicon-strip detectors became ubiquitous. Gaseous tracking in a variety of forms, such as TPCs and jet chambers, reached new levels of size and performance. There were also some notable firsts. The DELPHI collaboration developed the Ring Imaging Cherenkov Counter, a delicate technology in which the distribution of Cherenkov photons, imaged with mirrors onto photon-sensitive MWPC-type detectors, provides a measure of the particle’s velocity. The L3 collaboration aimed at ultimate-precision energy measurements of muons, photons and electrons, and put its money on a recently discovered scintillating crystal, bismuth germanate. Particle physicists, material scientists and crystallographers from academia and industry transformed this laboratory curiosity into mass-producible technology: ultimately, 12,000 crystals were grown, cut to size as truncated pyramids and assembled into the calorimeter, a pioneering trendsetter.
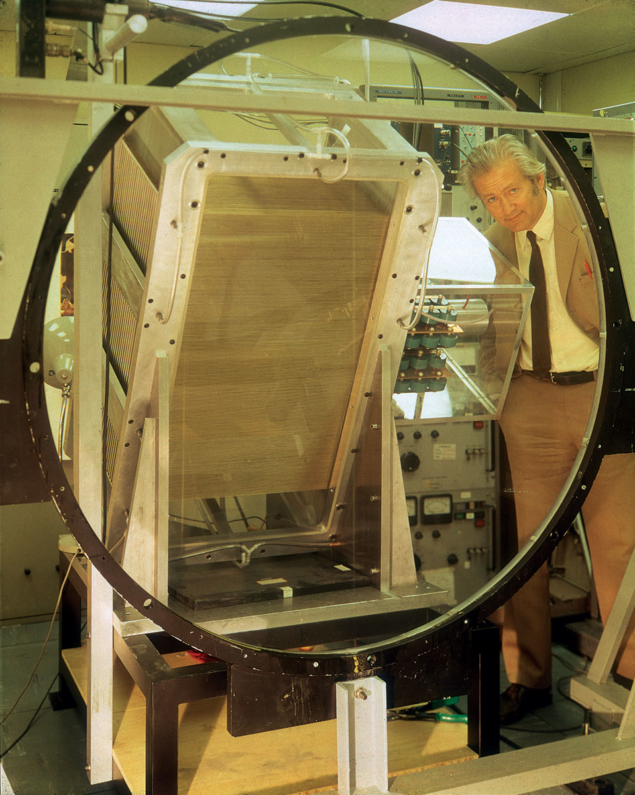
The ambition, style and success of these large, global collaborations was contagious. It gave the cosmic-ray community a new lease of life. The Pierre Auger Observatory, one of whose initiators was particle physicist and Nobel Laureate James Cronin, explores cosmic rays at extreme energies with close to 2000 detector stations spread over an area of 3000 km2. The IceCube collaboration has instrumented around a cubic kilometre of Antarctic ice to detect neutrinos. One of the most ambitious experiments is the Alpha Magnetic Spectrometer, hosted by the International Space Station – again with a particle physicist and Nobel Prize winner, Samuel Ting, as a prime mover and shaker.
These decade-long efforts in experimentation find their present culmination at the LHC. Experimenters had to innovate on several fronts: all detector systems were designed for and had to achieve ultimate performance, limited only by the laws of physics; the detectors must operate at a GHz or more collision rate, generating some 100 billion particles per second. “Impossible” was many an expert’s verdict in the early 1990s. The successful collaboration with industry giants in the IT and electronics sectors was a life-saver; and achieving all this – fraught with difficulties, technical and sociological – in international collaborations of several thousand scientists and engineers was an immense achievement. All existing detection technologies – ranging from silicon-tracking, to transition-radiation and RICH detectors, liquid-argon, scintillator and crystal calorimeters to 10,000 m3-scale muon spectrometers – needed novel ideas, major improvements and daring extrapolations. The success of the LHC experiments is beyond the wildest dreams: hundreds of measurements achieve a precision, previously considered only possible at electron–positron colliders. The Higgs boson, discovered in 2012, will be part of the research agenda for most of the 21st century, and CERN is in the starting block with ambitious plans.
Sharing with society
Worldwide, more than 30,000 accelerators are in operation. Particle and nuclear physics research uses barely more than 100 of them. Society is the principal client, and many of the accelerator innovations and particle detectors have found their way into industry, biology and health applications. A class of accelerators, to which CERN has contributed significantly, is specifically dedicated to tumour therapy. Particle detectors have made a particular impact on medical imaging, such as positron emission tomography (PET), whose origin dates back to CERN with a MWPC-based detector in the 1970s. Today’s clinical PETs use crystals, very similar to those used in the discovery of the Higgs boson.
Possibly the most important benefit of particle physics to society is the collaborative approach developed by the community, which underpins the incredible success that has led us to the LHC experiments today. There are no signs that the rate of innovation in detectors and instrumentation is slowing. Currently the LHC experiments are undergoing major upgrades and plans for the next generation of experiments and colliders are already well under way. These collaborations succeed in being united and driven by a common goal, bridging cultural and political divides.