Cosmological discoveries shed new light on the nature of fundamental particles, and vice versa. This synergy between the greatest and the smallest components of the universe was the subject of the 2004 DESY Theory Workshop, held in Hamburg.
There are a number of astrophysical phenomena, notably in connection with cosmology and ultrahigh-energy cosmic rays, that open a new window onto particle physics and lead to a better microscopic understanding of matter, space and time. On the other hand, particle physics is often exploited to great depths for an ultimate understanding of astrophysical phenomena, in particular the structure and evolution of the universe. These frontier-physics issues attracted a record number of 188 participants to Hamburg for the latest annual DESY Theory Workshop, held on 28 September – 1 October 2004 and organized by Georg Raffelt.
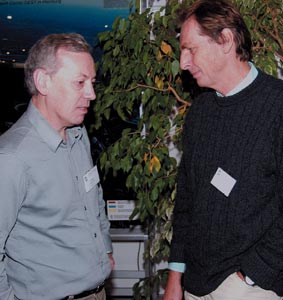
The workshop started with the traditional day of introductory lectures aimed at young physicists, which covered the main topics of the later plenary sessions. Most of the participants jumped at this opportunity. At the end of the day, they had learned much about Big Bang cosmology, including the thermal history of the universe; about the evolution of small fluctuations in the early universe, and their imprints on the cosmic microwave background (CMB) radiation and the large-scale distribution of matter; and about how these initial fluctuations may emerge during an inflationary era of the universe. They were also up to date in ultrahigh-energy cosmic-ray physics. Thus the ground was laid for the workshop proper.
Highlighting the dark
In recent years, significant advances have been made in observational cosmology, as several plenary talks emphasized. Observations of large-scale gravity, deep-field galaxy counts and Type Ia supernovae favour a universe that is currently about 70% dark energy – accounting for the observed accelerating expansion of the universe – and about 30% dark matter. The position of the first Doppler peak in recent measurements of the CMB radiation, by for example the Wilkinson Microwave Anisotropy Probe (WMAP) satellite, strongly suggests that the universe is spatially flat. These values for the cosmological parameters, together with today’s Hubble expansion rate, are collectively known as the “concordance” model of cosmology, for they fit a wide assortment of cosmological data. Indeed, we have entered the era of precision cosmology, with the precision set to continue increasing in the coming decade as a result of further observational efforts. It is now the turn of theoretical particle physicists to explain these cosmological findings, in particular why the dominant contribution to the energy density of the present universe is dark and what it is made of microscopically.
Dark matter
Successful Big Bang nucleosynthesis requires that about 5% of the energy content of the universe is in the form of ordinary baryonic matter. But what about the remaining non-baryonic dark matter?
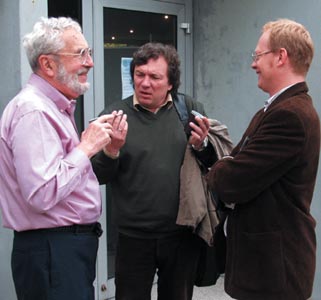
This 25% cannot be accounted for in the Standard Model of particle physics: the only Standard Model candidates for dark matter, the light neutrinos, were relativistic at the time of recombination and therefore cannot explain structure formation on small galactic scales. Studies of the formation of structure – as observed today by the Sloan Digital Sky Survey, for example – from primordial density perturbations measured in the CMB radiation yield an upper bound of about 2% on the energy fraction in massive neutrinos. This translates into an upper bound of around 1 eV for the sum of the neutrino masses. Observations, by means of the forthcoming Planck satellite, of distortions in the temperature and polarization of the CMB will improve the sensitivity in the sum of neutrino masses by an order of magnitude to 0.1 eV. This is comparable to the sensitivity of the future Karlsruhe Tritium Neutrino Experiment (KATRIN), which measures the neutrino mass via the tritium beta-decay endpoint spectrum, and of the planned second-generation experiments on neutrinoless double beta-decay.
In theories beyond the Standard Model, there is no lack of candidates for the dominant component of dark matter. Notable viable candidates are the lightest supersymmetric partners of the known elementary particles, which arise in supersymmetric extensions of the Standard Model: the neutralinos, which are spin-½ partners of the photon, the Z-boson and the neutral Higgs boson, and the gravitinos, which are spin-½ partners of the graviton. Showing that one of these particles accounts for the bulk of dark matter would not only answer a key question in cosmology, but would also shed new light on the fundamental forces and particles of nature.

While ongoing astronomical observations will measure the quantity and location of dark matter to greater accuracy, the ultimate determination of its nature will almost certainly rely on the direct detection of dark-matter particles through their interactions in detectors on Earth. Second-generation experiments such as the Cryogenic Dark Matter Search II (CDMS II) and the Cryogenic Rare Event Search with Superconducting Thermometers II (CRESST II), which are currently being assembled, will provide a serious probe of the neutralino as a dark-matter candidate.
Complementary, but indirect, information can be obtained from searches for neutrinos and gamma rays from neutralino-antineutralino annihilation, coming from the direction of particularly dense regions of dark matter, for example in the central regions of our galaxy, the Sun or the Earth. Ultimately, however, the proof of the existence of dark matter and the determination of its particle nature will have to come from searches at accelerators, notably CERN’s Large Hadron Collider (LHC). Even the gravitino, which is quite resistant to detection in direct and indirect dark-matter searches because it interacts only very feebly through the gravitational force, can be probed at the LHC.
Dark energy
In contrast with dark matter, dark energy has so far no explanation in particle physics. Apart from the observed accelerated expansion, the fact that we seem to be living at a special time in cosmic history, when dark energy appears only recently to have begun to dominate dark and other forms of matter, is also puzzling. Explanations put forth for dark energy range from the energy of the quantum vacuum to the influence of unseen space dimensions. Popular explanations invoke an evolving scalar field, often called “quintessence”, with an energy density varying in time in such a way that it is relevant today. Such an evolution may also be linked to a time variation of fundamental constants – a hot topic in view of recent indications of shifts in the frequencies of atomic transitions in quasar absorption systems, which hint that the electromagnetic fine-structure constant was smaller 7-11 billion years ago than it is today.
Depending on the nature of dark energy, the universe could continue to accelerate, begin to slow down or even recollapse. If this cosmic speed-up continues, the sky will become essentially devoid of visible galaxies in only 150 billion years. Until we understand dark energy, we cannot comprehend the destiny of the universe. Determining its nature may well lead to important progress in our understanding of space, time and matter.
The first order of business is to establish further evidence for dark energy and to discern its properties. The gravitational effects of dark energy are determined by its equation of state, i.e. the ratio of its pressure to its energy density. The more negative its pressure, the more repulsive the gravity of the dark energy. The dark energy influences the expansion rate of the universe, which in turn governs the rate at which structure grows, and the correlation between redshift and distance. Over the next two decades, high-redshift supernovae, counts of galaxy clusters, weak-gravitational lensing and the microwave background will all provide complementary information about the existence and properties of dark energy.
Inflationary ideas
The inflationary paradigm that the very early universe underwent a huge and rapid expansion is a bold attempt to extend the Big Bang model back to the first moments of the universe. It uses some of the most fundamental ideas in particle physics, in particular the notion of a vacuum energy, to answer many of the basic questions of cosmology, such as “Why is the observed universe spatially flat?” and “What is the origin of the tiny fluctuations seen in the CMB?”.
The exact cause of inflation is still unknown. Thermalization at the end of the inflationary epoch leads to a loss of details about the initial conditions. There is, however, a notable exception: inflation leaves a telltale signature of gravitational waves, which can be used to test the theory and distinguish between different models of inflation. The strength of the gravitational-wave signal is a direct indicator of what caused inflation. Direct detection of the gravitational radiation from inflation might be possible in the future with very-long-baseline, space-based, laser-interferometer gravitational-wave detectors. A promising shorter-term approach is to search for the signature of these gravitational waves in the polarized radiation from the CMB.
Matter Matters
The ordinary baryonic matter of which we are made is the tiny residue of the annihilation of matter and antimatter that emerged from the earliest universe in not-quite-equal amounts. This tiny imbalance may arise dynamically from a symmetric initial state if baryon number is not conserved in interactions that violate the conservation of C (C = charge conjugation) and the combination CP (P = parity), which produce more baryons than antibaryons in an expanding universe.
There are a few dozen viable scenarios for baryogenesis, all of which invoke more or less physics beyond the Standard Model. A particularly attractive scenario is leptogenesis, according to which neutrinos play a central role in the origin of baryon asymmetry. Leptogenesis predicts that the out-of-equilibrium, lepton-number violating decays of heavy Majorana neutrinos, with an exchange responsible for the smallness of the masses of the known light neutrinos, generate a lepton asymmetry in the early universe that is transferred into a baryon asymmetry by means of non-perturbative electroweak baryon- and lepton-number violating processes. Leptogenesis works nicely within the currently allowed window for the masses of the known light neutrinos.
Heavenly accelerators
The Earth’s atmosphere is continuously bombarded by cosmic particles. Ground-based observatories have measured them in the form of extensive air showers with energies up to 3 x 1020 eV, corresponding to centre-of-mass energies of 750 TeV, far beyond the reach of any accelerator here on Earth. We do not yet know the sources of these particles and thus cannot understand how they are produced.
Astrophysical candidates for high-energy sources include active galaxies and gamma-ray bursts. Alternatively, a completely new constituent of the universe could be involved, such as a topological defect or a long-lived superheavy dark-matter particle, both associated with the physics of grand unification. Only by observing many more of these particles, including the associated gamma rays, neutrinos and perhaps gravitational waves, will we be able to distinguish these possibilities.
Identifying the sources of ultrahigh-energy cosmic rays requires several kinds of large-scale experiments, such as the Pierre Auger Observatory, currently under construction, to collect large enough data samples and determine the particle directions and energies precisely. Dedicated neutrino telescopes of cubic-kilometre size in deep water or ice, such as IceCube at the South Pole, can be used to search for cosmic sources of high-energy neutrinos. An extension of their sensitivity to the ultrahigh-energy regime above 1017 eV will offer possibilities to infer information about physics in neutrino-nucleon scattering beyond the reach of the LHC.
Further reading
The Committee on the Physics of the Universe, Board on Physics and Astronomy, National Research Council 2003 Connecting Quarks with the Cosmos: Eleven Science Questions for the New Century (The National Academies Press, Washington, DC).