During 1998-9, Peter Kalmus of London’s Queen Mary and Westfield College lectured on “Particles and the universe” in 43 UK locations to 10 000 high-school students.Readers of CERN Courier will be familiar with the particle physics aspects of his story. A version of the first part, dealing with the universe, is published here.
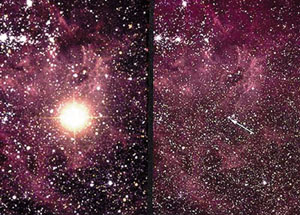
On 23 February 1987 an explosion that was a billion billion billion times as powerful as a hydrogen bomb was detected on Earth. It was Supernova 1987A, the first exploding star visible to the naked eye since the one that was detected by Kepler in 1604. The star, 170 000 light-years away in the Large Magellanic Cloud, ran out of nuclear fuel, collapsed under the influence of its own strong gravity and, in a few seconds, released a hundred times as much energy as our Sun has poured out in its entire lifetime.
However, even before a Canadian astronomer on a mountain in Chile first noticed the light of Supernova 1987A, ghostly messengers called neutrinos were registered in two huge underground particle detectors in the US and Japan. These detectors, consisting of a few thousand tonnes of very pure water, equipped with photomultipliers and electronics, had been built for a quite different purpose. They were designed to check whether protons were stable or whether they might undergo a very slow radioactive decay. No proton decays have yet been seen, but the detection of supernova neutrinos gave information both about these particles and about stellar collapse, and it was a dramatic illustration of the interplay between astronomy and particle physics.
Of course, the biggest explosion of all was the Big Bang – the creation of the universe about 12 billion years ago. The early universe was incredible – a dense primordial soup of elementary particles, colliding repeatedly at tremendous energies – a brilliant fireworks display. Indeed, the present universe with all its beauty and complexity is merely the wisp of smoke remaining after the fireworks show.

Today’s particle physics allows us, in a way, to recreate some conditions of the early universe. Readers who have spent many hours learning history, spanning perhaps a mere few thousand years, may be pleased to see the history of the universe displayed on a rather simple graph. The temperature of the universe in Kelvin is plotted on the right y-axis against time in seconds on the bottom x-axis. Both axes are logarithmic. On the left y-axis is plotted the average energy per particle, which is proportional to the temperature. The energy-mass density of the universe is plotted on the top x-axis, in units of equivalent mass density relative to the density of terrestrial water.
The CERN LEP and Fermilab Tevatron colliders have energies of around 100 GeV per elementary constituent (quark or lepton), and such energies were normal when the universe had a temperature of 1015 K, around 10-11 s after the Big Bang. The constituent particles – even neutrinos – were in almost perfect equilibrium with each other. Annihilation and creation were in balance. The universe then, as now, contained vastly more photons than quarks, and the energies per quark or lepton were then much greater than the rest masses, so the universe was accurately described as “radiation dominated”. As the universe expanded, it stretched the wavelength of radiation so that the photons had lower energies. The concentration of elementary objects was also reduced, thus the universe cooled. As we follow this thermal history, a number of remarkable events occur, leading to our present world.
Annihilation
At around 10-6 s the average energy had dropped to a few giga electron-volts, and quarks could combine into hadrons, and a bit later into the stable protons and (relatively stable) neutrons. At around 1 s, although the density was still several hundred thousand times that of water, collisions of neutrinos became rare – they could no longer be in thermal equilibrium with other particles and effectively decoupled for ever from the rest of matter and radiation. After a few more seconds, when the energy dropped below the mega electron-volt level, electrons and positrons could no longer be created, so they annihilated, leaving just sufficient electrons to balance the charge of the protons.
Some of the protons and neutrons could combine into deuterons, and then alpha particles, before the density and collision rate became too low. Then any remaining unbound neutrons decayed in the following hours. The amounts of deuterium and helium, which can be measured today, are a sensitive test of the conditions at that time, and hence of the Big Bang model. Tiny traces of isotopes of lithium could have been formed, but the absence of stable nuclei of mass 5 and mass 8 prevented the creation of further nuclei. This nucleosynthesis occurred at around 3 min.
After some 300 000 years the temperature had dropped to around 104 K and the average energy to around 1 eV, below the ionization potential of atoms. Neutral atoms of hydrogen and helium were formed. Photons were no longer impeded by frequent interactions with matter (they couple to charged particles), and the universe, which had until then been opaque, became transparent. Photons thus decoupled from matter. The dominant energy density after this was in the form of matter (including dark matter, the nature of which has not yet been determined), having previously been in the radiation. However, the temperatures and energies shown in the diagram, even in the matter-dominated era, represent those of the radiation. With the expansion of the universe, this radiation has now cooled to 2.7 K, the cosmic microwave background (CMB).
Star material
Gravity, acting on density ripples that have now been detected as tiny anisotropies in the CMB, caused matter to form clumps, which later became galaxies and stars. Thus the objects of astronomy make their appearance in the bottom right corner of the diagram. The first stars were composed of hydrogen and helium only. Fusion processes and other nuclear reactions in the cores of stars created the remaining elements.
The more massive stars had shorter lifetimes, and some exploded as supernovae, thereby polluting the local cosmos with these chemicals, and contributing to the mixture of elements out of which later stars, including our Sun and its solar system, could be formed. Every carbon and heavier nucleus in the Earth and in our bodies was formed at the centre of some now exploded star. We are all made of star material!
Until recently, cosmological measurements were consistent with the Big Bang expansion, opposed by the attraction of gravity. Depending on the mean mass-energy density of the universe, this could lead to continuous expansion or to ultimate contraction: the Big Crunch. Most cosmological measurements were rough. A few years ago, measurements indicated that the age of the universe was a bit less than the age of some stars, but, because of the observational uncertainties, a factor of less than two did not cause undue concern.
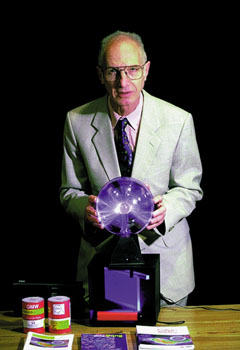
However, observations are getting much better. Results in the last year from two collaborations indicate that the expansion may actually be accelerating: very distant supernovae appear fainter than expected, indicating that they may be further away than implied from their redshifts. Careful checks on the supernova analyses are in progress, and additional distant supernovae are within the range of existing telescopes.
The observations can be explained by invoking Einstein’s cosmological constant – a kind of cosmic repulsion or negative vacuum pressure, which Einstein later regarded as his “biggest mistake”. Variations, such as “quintessence” – a fifth force that changes with time, – are also receiving attention. The energy density associated with a cosmological constant would affect the early structures in the universe and hence the angular anisotropy of the CMB – the ripples in the universe.
The CMB fluctuations are being measured with good precision at smaller angular scales, and their analyses show better consistency with supernovae and other observations if an additional cosmic repulsion is allowed. The energy density associated with the cosmological constant appears to be greater than that of matter. New CMB anisotropy results from the balloon-borne Boomerang project are about to be released, and these will be followed in the next few years by the NASA MAP and ESA Planck missions. The era of precision cosmology is about to begin, and its symbiosis with particle physics will result in more exciting science in the new century.