DARWIN observatory to be the ultimate dark-matter detector.
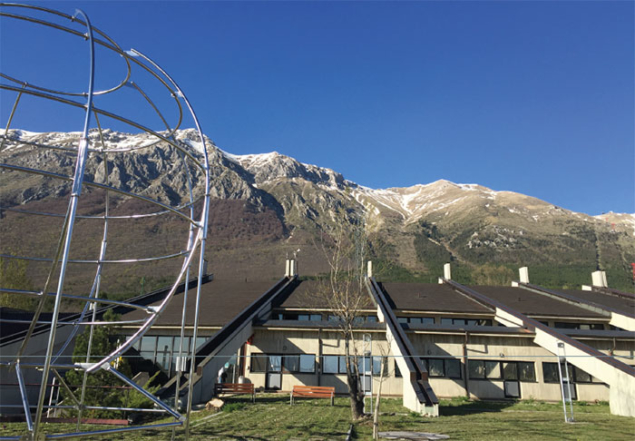
Image credit: L Baudis.
Dark matter is one of the greatest mysteries of our cosmos. More than 80 years after its postulation in modern form by the Swiss–American astronomer Fritz Zwicky, the existence of a new unseen form of matter in our universe is established beyond doubt. Dark matter is not just the gravitational glue that holds together galaxies, galaxy clusters and structures on the largest cosmological scales. Over the past few decades it has become clear that dark matter is also vital to explain the observed fluctuations in cosmic-microwave-background radiation and the growth of structures that began from these primordial density fluctuations in the early universe. Yet despite overwhelming evidence, its existence is inferred only indirectly via its gravitational pull on luminous matter. As of today, we lack the answer to the most fundamental questions: what is dark matter made of and what is its true nature?
DARWIN, the ultimate dark-matter detector using the noble element xenon in liquid form, will be in a unique position to address these fundamental questions. Currently in the design and R&D phase, DARWIN will be constructed at the Gran Sasso National Laboratory (LNGS) in Italy and is scheduled to carry out its first physics runs from 2024. The DARWIN consortium is growing, and currently consists of about 150 scientists from 26 institutions in 11 countries.
Worldwide search
The particles described by the Standard Model of particle physics are unable to account for dark matter. Although neutrinos, the only elementary particles that do not interact with photons, would be ideal candidates, they are much too light and do not form the observed large-scale structures. Dark matter could, however, be made of new elementary particles that were born in the young and energetic universe. Such particles would carry no electric or colour charge, would be either stable or very long-lived and, similar to neutrinos, would interact only feebly (if at all) with known matter via new fundamental forces. Theories beyond the Standard Model predict a wealth of viable dark-matter candidates. The most popular class has the generic name of weakly interactive massive particles (WIMPs), while a different class is axions, or more generally axion-like particles (ALPs).
Worldwide, more than a dozen experiments are preparing to observe low-energy nuclear recoils induced by galactic WIMPs in ultra-sensitive, low-background detectors. Since the predicted WIMP masses and scattering cross-sections are model-dependent and essentially unknown, searches must cover a vast parameter space. Among the most promising detectors are those based on liquefied noble-gas targets such as liquid xenon (LXe) or liquid argon (LAr) – a well-established technology that can be scaled up to tonne-scale target masses and take data over periods lasting several years.

DARWIN, which will operate a multi-tonne liquid-xenon time projection chamber (TPC), follows in the footsteps of its predecessors XENON, ZEPLIN, LUX and PandaX. The technology employed by these experiments is very similar and, in addition, the entire XENON collaboration is now a part of the DARWIN collaboration. Since December 2016, an upgraded detector called XENON1T has been recording its first dark-matter data at LNGS using two tonnes of liquid xenon as the WIMP target (the total mass of xenon in the detector is 3.3 tonnes). It will probe WIMP–nucleon cross-sections down to as little as 1.6 × 10–47 cm2 at a mass of 50 GeV/c2 (for comparison, the scattering cross-section of low-energy 7Be solar neutrinos on electrons is about 6 × 10–45 cm2). A further planned upgrade called XENONnT with seven tonnes of LXe will increase the WIMP sensitivity by one order of magnitude.
The goals of DARWIN are even more ambitious, promising an unprecedented sensitivity of 2.5 × 10–49 cm2 at a WIMP mass of 40 GeV/c2. Such a reach would allow us to explore the entire experimentally accessible parameter space for WIMPs, to the point where the WIMP signal becomes indistinguishable from background processes from coherent neutrino-nucleus scattering events.
Rich physics programme
DARWIN will not only search for WIMP dark matter. Because of its ultra-low background level, it will be sensitive to additional, hypothetical particles that are expected to have non-vanishing couplings to electrons. These include solar axions, galactic ALPs and bosonic super-weakly interacting massive particles called superWIMPs, which have masses at the keV scale and are candidates for warm dark matter. It will also detect low-energy solar neutrinos produced by proton–proton fusion reactions in the Sun (so-called pp neutrinos) with high statistics, and therefore address one of the remaining observational challenges in the field of solar neutrinos: a precise comparison of the Sun’s neutrino and photon luminosities. Capable of providing a statistical precision of less than one per cent on this comparison with just five years of data, the high-statistics measurement of the pp-neutrino flux would provide a stringent test of the solar model, as well as neutrino properties, because non-standard neutrino interactions could modify the survival probability of electron neutrinos at these low energies.
The DARWIN observatory will also observe coherent neutrino-nucleus interactions from 8B solar neutrinos and be sensitive to neutrinos of all flavours from core-collapse supernovae: it would see about 800 events, or 20 events/tonne, from a supernova with 27 solar masses at a distance of 10 kpc, for example. By looking at the time evolution of the event rate from a nearby supernova, DARWIN could possibly even distinguish between different supernova models. Finally, DARWIN would search for the neutrinoless double beta (0νββ) decay of 136Xe, which has a natural abundance of 8.9 per cent in xenon. The observation of this ultra-rare nuclear decay would directly prove that neutrinos are Majorana particles, and that lepton number is violated in nature (CERN Courier July/August 2016 p34).
One common feature of these exciting questions in contemporary particle and astroparticle physics is the exceedingly low expected interaction rates in the detector, corresponding to less than one event per tonne of target material and year. In addition, these searches – with the exception of the 0νββ decay – require an energy threshold that is as low as possible (a few keV), while the 0νββ decay, superWIMP and axion searches will profit from the very good energy resolution of the detector. A multi-tonne liquid-xenon observatory such as DARWIN can address the combination of an ultra-low background level, a low-energy threshold and a good energy resolution within a single, large, monolithic detector.
The WIMP landscape
The current best sensitivity to WIMP searches for masses above 6 GeV/c2 is provided by detectors using LXe as a target, and the majority of existing (XENON1T, LUX, PandaX) and planned (LZ, XENONnT) LXe dark-matter detectors employ dual-phase TPCs (figure 1). These detectors maintain xenon at a constant temperature of about –100 °C and detect two distinct signals (the prompt scintillation light and the ionisation electrons) via arrays of photosensors operated in the liquid and vapour phase. The observation of both signals delivers information about the type of interaction and its energy, as well as the 3D position and timing of an event. WIMP collisions and coherent neutrino scatters will produce nuclear recoils, while pp neutrinos, axions, superWIMPs and double beta decays, along with the majority of background events, will cause electronic recoils. Fast neutrons from materials or induced through cosmic-ray muons will also give rise to nuclear recoils, but WIMPs and neutrinos will scatter only once in a given detector, while neutrons can scatter multiple times in large detectors such as DARWIN.
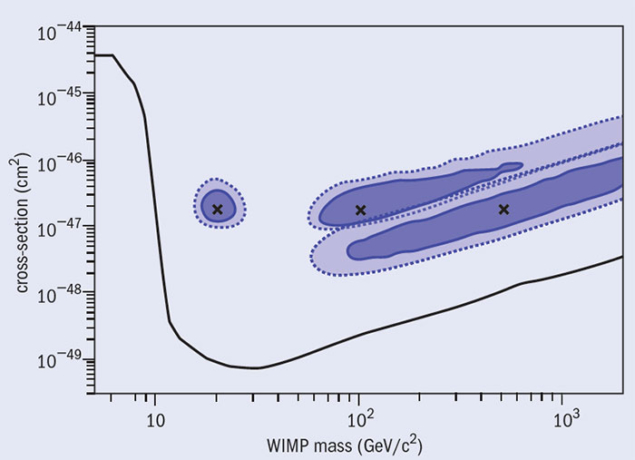
Since the primary intent of DARWIN is to investigate dark-matter interactions, it is vital that background processes are understood. The observatory can exploit the full discovery potential of the liquefied xenon technique with a 40 tonne LXe TPC that allows all known sources of background to be considered. These stem from several sources: the residual radioactivity of detector-construction materials (γ radiation, neutrons); β decays of the anthropogenic 85Kr present in the atmosphere due to nuclear fuel reprocessing, weapons tests and accidents such as that at Fukushima nuclear plant in Japan; and the progenies of 222Rn in the LXe target. Two-neutrino double beta decays (2νββ) of 136Xe and interactions of low-energy solar neutrinos (pp, 7Be) are another source of background, as are higher-energy neutrino interactions with xenon nuclei in coherent neutrino-nucleus scattering.
In the standard WIMP-scattering scenario, the leading interactions between a dark-matter particle and a nucleon are due to two subtly different processes: spin-dependent couplings and isospin-conserving, spin-independent couplings. Since LXe contains nuclei with and without spin, DARWIN can probe both types of interactions. Assuming an exposure of 200 tonnes × years (500 tonnes × years), a spin-independent WIMP sensitivity of 2.5 × 10–49 cm2 (1.5 × 10–49 cm2) can be reached at a WIMP mass of 40 GeV/c2. For spin-dependent WIMP–neutron couplings and WIMP masses up to about 1 TeV, the searches conducted by DARWIN will be complementary to those of the LHC and High-Luminosity LHC at a centre-of-mass energy of 14 TeV. Natural xenon includes two isotopes with nonzero total nuclear angular momentum, 129Xe and 131Xe, at a combined abundance of about 50%. If the WIMP–nucleus interaction is indeed spin-dependent, DARWIN will also probe inelastic WIMP–nucleus scattering, where these two nuclei are excited into low-lying states at 40 keV and 80 keV, respectively, with subsequent prompt de-excitation. The discovery of such a signature would be a clear indication for an axial-vector coupling of WIMPs to nuclei.
Ultimate detector
Should dark-matter particles be discovered by one of the running (XENON1T, DEAP-3600) or near-future (LZ, XENONnT) detectors, DARWIN would be able to reconstruct the mass and scattering cross-section from the measured nuclear recoil spectra. With an exposure of 200 tonnes × years, 152, 224 and 60 events would be observed for the three WIMP masses, respectively (figure 2). DARWIN may therefore be the ultimate liquid-xenon dark-matter detector, capable of probing the WIMP paradigm and thus detect or exclude WIMPs with masses above 6 GeV/c2, down to the extremely low cross-sections of 1.5 × 10–49 cm2.
Should WIMPs not be observed in the DARWIN detector, the WIMP paradigm would be under very strong pressure. With its large, uniform target mass, low-energy threshold, and ultra-low background level, the observatory will also open up a unique opportunity for other rare event searches such as axions and other weakly interacting light particles. It will address open questions in neutrino physics, which is one of the most promising areas in which to search for physics beyond the Standard Model. At its lowest energies, the DARWIN detector will observe coherent neutrino-nucleus interactions from solar 8B neutrinos, thus precisely testing the standard-solar-model flux prediction, and may detect neutrinos from galactic supernovae.
The DARWIN observatory was approved for an initial funding period, via ASPERA, in 2010. It is included in the European Roadmap for Astroparticle Physics and in various other programs, for example by the Swiss State Secretariat for Education, Research and Innovation and the Strategic Plan for Astroparticle Physics in the Netherlands. The current phase will culminate with a technical design report in 2019, followed by engineering studies in 2020 and 2021, with the construction at LNGS and first physics runs scheduled to start in 2022 and 2024, respectively. The experiment will operate for at least 10 years and may write a new chapter in the exciting story of dark matter.