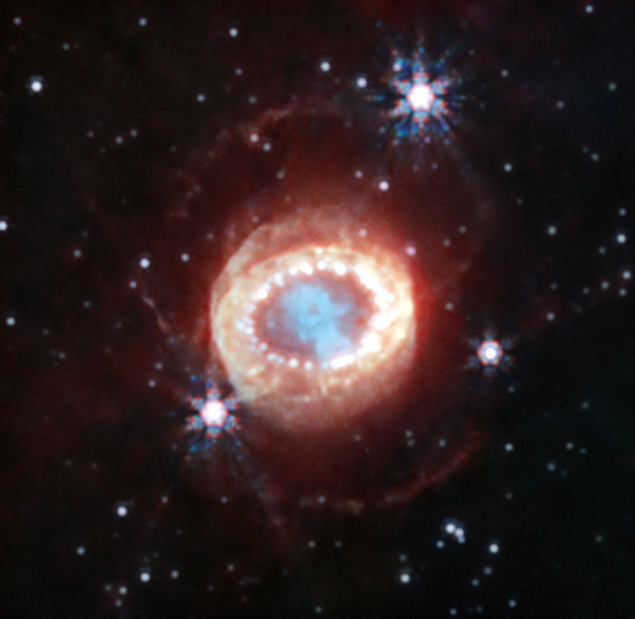
Towards the end of the lifetime of a very massive (>8 M☉) star, the nuclear fusion processes in its core are no longer sufficient to balance the constantly increasing pull of gravitational forces. This eventually causes the core to collapse, with the release of an enormous amount of matter and energy via shockwaves. Nearly 99% of such a core-collapse supernova’s energy is released in the form of neutrinos, usually leaving behind a compact proto-neutron star with a mass of about 1.5 M☉ and a radius of about 10 km. For more massive remnant cores (>3 M☉), a black hole is formed instead. The near-zero mass and electrical neutrality of neutrinos make their detection particularly challenging: when the famous 1987 supernova SN1987a occurred 168,000 light-years from Earth, the IMB observatory in the US detected just eight neutrinos, BNO in Russia detected 13 and Kamiokande II in Japan detected 11 (CERN Courier March/April 2021 p12).
Besides telling us about the astrophysical processes inside a core-collapse supernova, such neutrino detections might also tell us more about the particles themselves. The Standard Model (SM) predicts feeble self-interactions among neutrinos (νSI), but probing them remains beyond the reach of present-day laboratories on Earth. As outlined in a white paper published earlier this year by Jeffrey Berryman and co-workers, νSI (mediated, for example, by a new scalar or vector boson) enter many beyond-the-SM theories that attempt to explain the generation of neutrino masses and the origin of dark matter. One of the probes that can be used to explore such interactions are core-collapse supernovae, since the extreme conditions in these catastrophic events make it more likely for νSI to occur and therefore affect the behaviour of the emitted neutrinos.
Recently, Po-Wen Chang and colleagues at Ohio State University explored this possibility by considering the formation of a tightly coupled neutrino fluid that expands under relativistic hydrodynamics, thereby having an effect on neutrino pulses detected on Earth. The team derives solutions to relativistic hydrodynamic equations for two cases: a “burst outflow” and a “wind outflow”. A burst outflow of a uniform neutrino fluid occurs when it undergoes free expansion in vacuum, while a wind outflow occurs when steady-state solutions to the hydrodynamics equations are looked for. In their current work, the authors focus on the former.
In a scenario without νSI, the neutrinos escape and form a shell of thickness about 105 times the radius of the proto-neutron star that freely travels away at the speed of light. On the other hand, in a scenario with νSI, the neutrinos don’t move freely immediately after escaping the proto-neutron star and instead undergo increased neutrino elastic scattering. As a result, the neutrino shell continues expanding radially until it reaches the point where the density becomes low enough for the neutrinos to decouple and begin free-flowing. The thickness of the shell at this instant depends on the strength of the νSI interactions and is expected to be much larger than that in the no-νSI case. This, in turn, would translate to longer neutrino signals in detectors on Earth.
The effects of neutrino self interactions on SN1987a are starting to become clearer
Data from SN1987a, where the neutrino signal lasted for about 10 s, broadly agree with the no-νSI scenario and were used to set limits on very strong νSI interactions. On the other hand, if νSI were to exist as a burst-outflow, the proposed model gives very robust results, with an estimated sensitivity of 3 s. Additionally, the authors argue that the steady-state wind-outflow case might be more likely to occur, a dedicated treatment of which has been left for future work.
For the first time since its observation 36 years ago, the effects of νSI on SN1987a are starting to become clearer. Further advances in this direction are much anticipated so that when the next supernovae occurs it could help clear the fog that surrounds our current understanding of neutrinos.
Further reading
J Berryman et al. 2023 Phys. Dark Universe 42 101267.
P-W Chang et al. 2023 Phys. Rev. Lett. 131 071002.