Two prominent scientists recall the work and personality of the Nobel laureate who was their mentor.
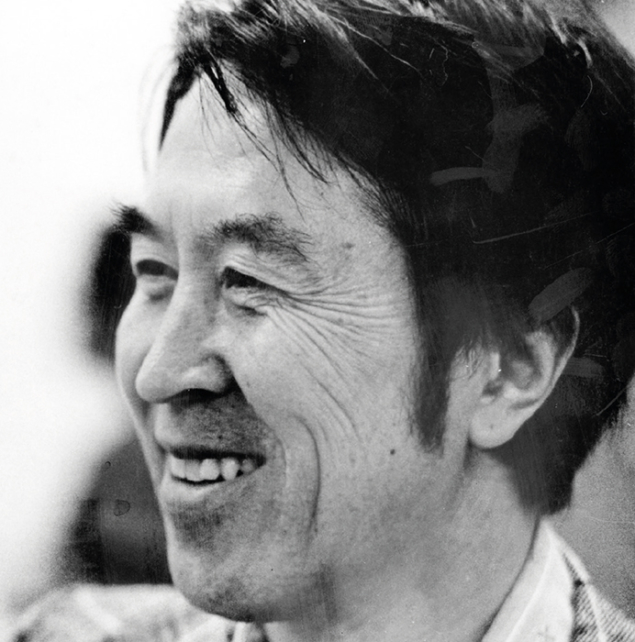
Image credit: Spenta Wadia.
Yoichiro Nambu passed away on 5 July 2015 in Osaka. He was awarded the Nobel Prize in Physics in 2008 “for the discovery of the mechanism of spontaneous broken symmetry in subatomic physics”. Nambu’s work in theoretical physics spanning more than half a century is prophetic, and played a key role in the development of one of the great accomplishments of 20th century physics – the Standard Model of particle physics. He was also among those who laid the foundations of string theory.
The early years
When Nambu graduated from the University of Tokyo in 1943, Japan was in the midst of the Second World War – but at the same time, Japanese physics was extremely vibrant. Among other things, a group of superb Japanese physicists were developing the framework of quantum field theory. This spark came from the work of Hideki Yukawa in the 1930s, who laid the foundations of modern particle physics by his prediction that the force between nucleons inside a nucleus is caused by the exchange of a particle (today called the pion) that, unlike the photon, had a mass. Yukawa showed that this results in a force that dies out quickly as the distance between the nucleons is increased, as opposed to electromagnetic forces, caused by a massless photon, which have infinite range. Yukawa was Japan’s first Nobel laureate, in 1949. Soon afterwards, Japan became a powerhouse of particle physics and quantum field theory. In 1965, Sin-Itiro Tomonaga received the Nobel prize (shared with Richard Feynman and Julian Schwinger) for his work on the quantum field theory of electromagnetism.
In 1948, Nambu joined a select group of theoretical physicists at the newly formed department at Osaka City University. He spent three formative years there: “I had never felt and enjoyed so much the sense of freedom.” Much of his early work dealt with quantum field theory. One influential paper dealt with the derivation of the precise force laws in nuclear physics. In the process, he derived the equation that describes how particles can bind with each other – an equation that was later derived independently by Bethe and Salpeter, and is now known commonly as the Bethe–Salpeter equation.
Nambu always felt that his work in physics was guided by a philosophy – one that was uniquely his own. During his years in Osaka, he was deeply influenced by the philosophy of Sakata and Taketani. Sakata was yet another prominent theoretical physicist in Japan at that time: he later became well known for the Sakata model, which was a precursor to the quark model of nuclear constituents. Sakata was influenced by Marxist philosophy, and together with Taketani developed a “three-stage methodology” in physics. As Nambu recalled later, Taketani used to visit the young group of theorists at Osaka and “spoke against our preoccupation with theoretical ideas, emphasised to pay attention to experimental physics. I believe that this advice has come to make a big influence on my attitude towards physics”. Together with colleagues Nishijima and Miyazawa, he immersed himself in understanding the properties of the newly discovered elementary particles called mesons.
In 1952, J R Oppenheimer invited Nambu to spend a couple of years at the Institute of Advanced Study in Princeton. By his own account, this was not a particularly fruitful period: “I was not very happy.” After a summer at Caltech, he finally came to the University of Chicago at the invitation of Marvin Goldberger. There he became exposed to a remarkably stimulating intellectual atmosphere, which epitomised Fermi’s style of “physics without boundaries”. There was no “particle physics” or “physics of metals” or “nuclear physics”: everything was discussed in a unified manner. Nambu soon achieved a landmark in the history of 20th century physics: the discovery that a vacuum can break symmetries spontaneously. And he came up with the idea while working in a rather different area of physics: superconductivity.
Symmetries of the laws of nature often provide guiding principles in physics. An example is “rotational symmetry”. Imagine yourself to be in deep space, so far away from any star or galaxy that all you can see in any direction is empty space. Things look completely identical in all directions – in particular, if you are performing an experiment, the results would not depend on if you rotated your lab slowly and did the same thing. It is this symmetry that leads to the conservation of angular momentum. Of course, the rotational symmetry is only approximate, because there are stars and galaxies that break this symmetry explicitly.
There are other situations, however, where a symmetry is broken spontaneously. One example is a magnet. The molecules inside a magnet are themselves little magnetic dipoles. If we switch on a small magnetic field, then the rotational symmetry is broken explicitly and all of the dipoles align themselves in the direction of the magnetic field. That is simple. The interesting phenomenon is that the dipoles continue to be aligned in the same direction, even after the external magnetic field is switched off. Here the rotational symmetry is broken spontaneously.
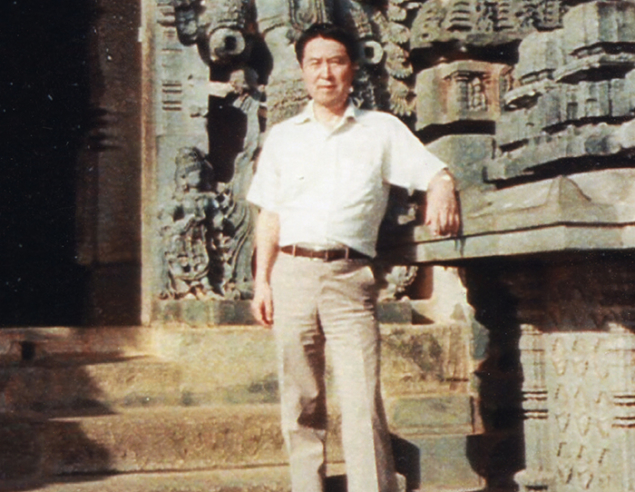
Image credit: Spenta Wadia.
Nevertheless, the fact that the underlying laws respect rotational symmetry has a consequence: if we gently disturb one of the dipoles from its perfectly aligned position, it gently nudges its neighbours and they nudge their neighbours, and the result is a wave that propagates through the magnet. Such a wave has very low energy and is called a spin wave. This is a special case of a general phenomenon where a spontaneously broken symmetry has an associated low-energy mode, or in quantum theory an associated massless particle.
Breaking symmetry
Nambu took the concept of spontaneous symmetry breaking to a new level. He came up with this idea while trying to understand the Bardeen–Cooper–Schrieffer (BCS) theory of superconductivity. Superconductors are materials that conduct electric current without any resistance. Superconductors also repel external magnetic fields – an effect called the Meissner effect. Inside a superconductor, electromagnetic fields are short-ranged rather than long-ranged: as if the photon has acquired a mass, like Yukawa’s mesons. However, a massive photon appears to be inconsistent with gauge invariance – a basic property of electromagnetism.
It was Nambu in 1959, and independently Philip Anderson a little earlier in 1958, who understood what was going on. They realised that (in the absence of electromagnetic interactions) the superconducting state broke the symmetry spontaneously. This symmetry is unlike the rotation symmetry that is spontaneously broken in magnets or crystals. It is a symmetry associated with the fact that electric charge is conserved. Also, if we imagine switching off the electromagnetic interaction, this symmetry breaking would also result in very low-energy waves, like spin waves in a magnet – a massless particle. Now comes a great discovery: if we switch on the electromagnetic interaction, which is there, we can undo the apparent symmetry breaking by a gauge transformation, which is local in space (and time), without any energy cost. Hence, there is no massless particle, and in fact the photon becomes massive together with a massive neutral particle, which explains the Meissner effect. The neutral scalar excitation in superconductors was discovered 20 years after it was predicted. This effortless excursion across traditional boundaries of physics characterised Nambu’s work throughout his career.
Soon after finishing his work on superconductivity, Nambu returned to particle physics. The first thing he noticed was that the Bogoliubov equations describing excitations near the Fermi surface in a superconductor are very similar to the Dirac equation that describes nucleons. The energy gap in a superconductor translates to the mass of nucleons. The charge symmetry that is spontaneously broken in a superconductor (electromagnetism switched off) also has an analogue – chiral symmetry. If the energy gap in a superconductor is a result of spontaneous symmetry breaking of charge symmetry, could it be that the mass of a nucleon is the result of spontaneous symmetry breaking of chiral symmetry? Unlike the charge symmetry in a superconductor, chiral symmetry is a global symmetry that can be truly spontaneously broken, leading to a massless particle – which Nambu identified with the pion. This is exactly what Nambu proposed in a short paper in 1960, soon followed by two papers with Jona-Lasinio.
This was a revolutionary step. In all previous examples, spontaneous symmetry breaking happened in situations where there were constituents (the molecular dipoles in a magnet, for example) and the underlying laws did not permit them to arrange themselves maintaining the symmetry. Nambu, however, proposed that there are situations where spontaneous symmetry breaking can happen in the vacuum of the world.
In physics, vacuum is the name given to “nothing”. How can a symmetry be broken – even spontaneously – when there is nothing around? The radical nature of this idea has been best described by Phil Anderson: “To me – and perhaps more to his fellow particle theorists – this seemed like a fantastic stretch of imagination. The vacuum, to us, was and always had been a vacuum – it had, since Einstein got rid of the aether, been the epitome of emptiness…I, at least, had my mind encumbered with the idea that if there was a condensate, there was something there…This is why it took a Nambu to break the first symmetry.”
Nambu was proposing that the masses of elementary particles have an origin – something we can calculate. The revolutionary nature of this idea cannot be overstated. Soon after the papers of Nambu and Jona-Lasinio, Goldstone came up with a simpler renormalisable model of superconductivity, which also illustrates the phenomenon of spontaneous symmetry breaking by construction and provided a general proof that such symmetry breaking always leads to a massless particle.
Meanwhile, in 1963 Anderson realised that the mechanism of generating masses for gauge particles that was discovered in superconductivity could be useful in elementary particle physics in the context of the nature of “vacuum of the world”. The mechanism was subsequently worked out in full generality by three independent groups, Higgs, Englert and Brout, and Guralnik, Hagen and Kibble, and is called the “Higgs mechanism”. It became the key to formulating the Standard Model of particle physics by Weinberg and Salam, building on the earlier work of Glashow, and resulting in our current understanding of electromagnetic and weak forces. The analogue of the special massive state in a superconductor is the Higgs particle, discovered at CERN in 2012.
We now know, for certain, that chiral symmetry is spontaneously broken in strong interactions. However, the final realisation of this idea had to wait until another work by Nambu.
The idea that all hadrons (particles that experience strong forces) are made of quarks was proposed by Gell-Mann, and independently Zweig, in 1964. However, the idea soon ran into serious trouble.
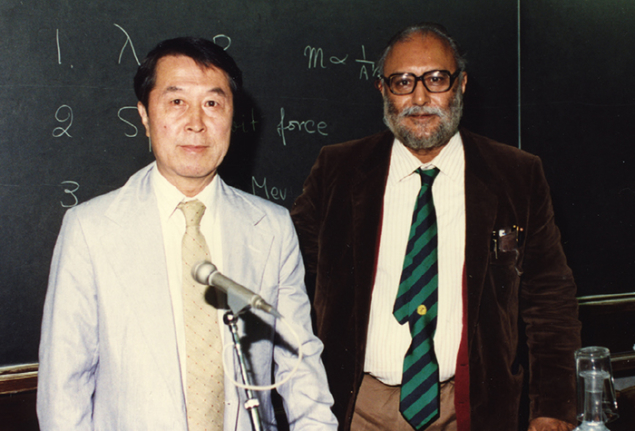
Image credit: Spenta Wadia.
Now, the quarks that make up nucleons have spin ½. According to the spin-statistics theorem, they should be fermions obeying the exclusion principle. However, it appeared that if quarks are indeed the constituents of all hadrons, they cannot at the same time be fermions. To resolve this contradiction, Nambu proposed that quarks possess an attribute that he called “charm” and is now called colour. In his first proposal, quarks have two such colours. Subsequently, in a paper with M Y Han, he proposed a model with three colours. Two quarks may appear identical (and therefore cannot be on top of each other) if their colour is ignored. However, once it is recognised that their colours are different, they cease to be identical, and the usual “exclusion” of fermions does not apply. A little earlier, O Greenberg came up with another resolution: he postulated that quarks are not really fermions but something called “para-fermions”, which have unconventional properties that are just right to solve the problem.
However, it was Nambu’s proposal that turned out to be more fruitful. This is because he made another remarkable one: colour is like another kind of electric charge. A quark not only produced an ordinary electric field, but a new kind of generalised electric field. This new kind of electric field causes a new kind of force between quarks, and the energy is minimum when the quarks form a colour singlet. This force, Nambu claimed, is the basic strong force that holds the quarks together inside a nucleon. This proposal turned out to be essentially correct, and is now known as quantum chromodynamics (QCD). In the model of Han and Nambu, quarks carry integer charges, which we now know is incorrect. In 1972, Fritzsch and Gell-Mann wrote down the model with correct charge assignments and proposed that only colour singlets occur in the spectrum, which would ensure that fractionally charged quarks remain unobserved. However, it was only after the discovery by David Gross, Frank Wilczek, and David Politzer in 1973 of “asymptotic freedom” for the generalised electric field that QCD became a candidate theory of the strong interactions. It explained the observed scaling properties of the strong interactions at high energies (which probe short distances) and indicated that the force between quarks had a tendency to grow as they were pulled apart.
Simple dynamical principle
String theory, which is recognised today as the most promising framework of fundamental physics including gravity, had its origins in making sense of strongly interacting elementary particles in the days before the discovery of asymptotic freedom. To make a long story short, Nambu, Nielsen and Susskind proposed that many mathematical formulae of the day, which originated from Veneziano’s prescient formula, could be explained by the hypothesis that the underlying physical objects were strings (one-dimensional objects) rather than point particles. This was a radical departure from the “Newtonian” viewpoint that elementary laws of nature are formulated in terms of “particles” or point-like constituents.
Nambu (and independently Goto) also provided a simple dynamical principle with a large local symmetry for consistent string propagation. His famous paper on the string model entitled “Duality and hadrodynamics” was submitted to the Copenhagen High Energy Physics Symposium in 1970. In a letter dated 4 September 1986, to one of us (SRW), Nambu wrote: “In August 1970, there was a symposium to be held in Copenhagen just before a High Energy Physics Conference in Kiev, and I was planning to attend both. But before leaving for Europe, I set out to California with my family so that they could stay with our friends during my absence. Unfortunately our car broke down as we were crossing the Great Salt Lake Desert, and we were stranded in a tiny settlement called Wendover for the three days. Having missed the flight and the meeting schedules, I cancelled the trip in disgust and had a vacation in California instead. The manuscript, however had been sent out to Copenhagen, and survived.”
It is quite common for scientists to become excessively attached to their own creations. In contrast, Nambu was remarkably open-minded. To him, his work was like placing a few pieces into a giant jigsaw puzzle: he never thought that he had discovered the “ultimate truth”. This deep sense of modesty was also a part of his personality. To the entire community of physicists, he was this shy, unassuming man, often difficult to understand, coming up with one original idea after another. There was a sense of play in the way that he did science: maybe that is why his ideas were sometimes incomprehensible when they first appeared.
Nambu’s legacy, “physics without boundaries”, must have had a subconscious influence on some of us in India involved in setting up the International Centre for Theoretical Sciences (ICTS), a centre of TIFR in Bangalore, where “science is without boundaries”.
We end with a quote from Nambu’s speech at the Nobel presentation ceremony at the University of Chicago on 10 December 2008, which clearly shows his view of nature: “Nowadays, the principle of spontaneous symmetry breaking is the key concept in understanding why the world is so complex as it is, in spite of the many symmetry properties in the basic laws that are supposed to govern it. The basic laws are very simple, yet this world is not boring; that is, I think, an ideal combination.”
• An earlier version of the article appeared in Frontline magazine, see www.frontline.in/other/obituary/a-giant-of-physics/article7593580.ece.