The discovery of large-scale features in the gamma-ray sky in approximately the same direction continues to puzzle researchers, calling for multi-wavelength observations with the next generation of telescopes.
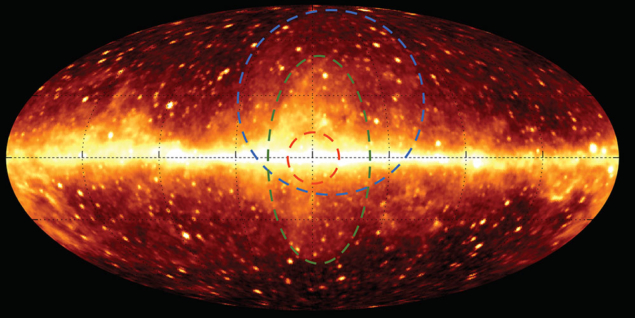
High-energy gamma rays provide a window into the physics of cosmic objects at extreme energies, such as black holes, supernova remnants and pulsars. In addition to revealing the nature of such objects, high-energy gamma-ray signals test general relativity and the Standard Model of particle physics. Take for example gamma-ray bursts, which can last from 10 milliseconds to several hours and are emitted by sources located up to several billion light-years away from Earth. A comparison between the arrival times of the bursts’ X-rays and gamma rays has been used to exclude modifications of Einstein’s general relativity that predict different arrival times. Also, in some theories in which dark matter is in the form of weakly interacting massive particles (WIMPs), dark-matter particles can annihilate into gamma-ray photons and other Standard Model particles. Significant effort is therefore being spent in searches for dark-matter annihilation signals in the gamma-ray band, including searches towards the Milky Way centre, which is estimated to contain a large amount of dark matter.
Studies of individual gamma-ray emitting sources and diffuse gamma-ray emission, which could include a galactic dark-matter annihilation signal, have benefited greatly from the launch of the large-area telescope on board NASA’s Fermi Gamma-ray Space Telescope (Fermi-LAT) in June 2008. Fermi-LAT, which observes gamma rays with energies from about 20 MeV to 1 TeV, has discovered more than 3000 point sources that have enabled researchers to significantly improve models of known galactic and extragalactic gamma-ray-emitting objects. But Fermi-LAT has also thrown up some surprising discoveries (figure 1). One of these is the so-called Fermi bubbles – two large gamma-ray lobes above and below the galactic centre that, intriguingly, have no clear counterpart in the X-ray and radio bands.
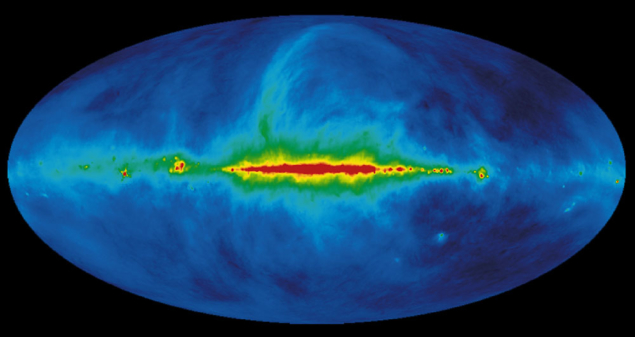
A second unexpected discovery by Fermi-LAT was an excess of gamma-ray radiation near the galactic centre with an energy of a few GeV. Interestingly, the excess has properties that are consistent with an annihilation signal from dark-matter particles with a mass of a few tens of GeV. The excess is visible up to 10 or 15 degrees away from the galactic centre – an elephant at a distance of four metres from an observer would have a similar apparent size. The Fermi bubbles, spanning 110 degrees from the northern to the southern edge, have an apparent size comparable to that of an elephant located one metre away.

Finally, there is a third, even larger, feature in the gamma-ray, radio and X-ray bands called Loop I. The challenge of explaining these three “elephants” in the gamma-ray sky has puzzled physicists and astronomers for years – tens of years in the case of Loop I. Are the features related to each other? Are they located near the galactic centre or close to us? And is the GeV gamma-ray excess caused by dark-matter annihilation or by astrophysical phenomena such as pulsars?
Loop I
The largest of the gamma-ray elephants, Loop I, has been known since the 1950s from its radio emission (figure 2a). Its large angular size – it stretches up to 80 degrees above the galactic plane – could easily be explained if it were a nearby feature. For instance, it could be the combined emission from a “superbubble”, the collective remnant of several supernova explosions taking place in a localised region. Such a bubble easily reaches a size of a few hundred light-years, and if the distance to the bubble was also a few hundred light-years, then it would appear very large, up to 90 degrees in angular size. In this scenario, the galactic magnetic field would drape around the expanding bubble and high-energy cosmic-ray electrons from sources in the galactic disk, compressed by the expansion of the bubble, would produce synchrotron emission that would appear as a huge, ring-like structure in the sky. A possible location of the underlying supernova explosions would be the Scorpius–Centaurus stellar cluster located a few hundred light-years away from Earth.
Loop I, or at least its brightest part, known as the North Polar Spur, is also seen at other wavelengths, in particular at gamma-ray (figure 1) and soft X-ray (figure 2b) wavelengths. While the gamma rays can be produced through inverse Compton emission by the same cosmic-ray electrons that produce the synchrotron radio emission, the soft X-ray emission is probably produced by hot interstellar gas. The approximate angular alignment between the radio and X-ray emissions of the North Polar Spur suggests that they both belong to Loop I. Yet there are several differences between the X-ray and radio emissions. For example, a bright, ring-like feature in X-rays that is crossing the North Polar Spur could be explained by the collision of the hypothetical Loop I superbubble with another bubble containing the solar system, the local hot bubble. One can even trace back the motion of stars within a few hundred light-years from us to find a population of stars with members that could have exploded as supernovae up to about 10 million years ago and inflated the local hot bubble.
However, apparent X-ray absorption at the southern part of the North Polar Spur by neutral gas located along the line of sight points to a different interpretation. Detailed spectral modelling of this absorption has recently shown that the amount of gas required to explain the absorption puts the X-ray emitting structure at distances far beyond a few hundred light-years. This lower bound on the distance to the X-ray structure favours models of Loop I as a galactic-scale phenomenon, for example the product of a large-scale outflow from the galactic-centre region, as opposed to the nearby superbubble. More X-ray data is needed to pin down the nature of Loop I, but if this feature is indeed a large-scale galactic structure, then it might be related to the second elephant in the sky – the Fermi bubbles.
Fermi bubbles
The Fermi bubbles consist of two large gamma-ray lobes above and below the galactic centre, each of which is slightly larger than the distance from Earth to the galactic centre (about 25,000 light-years). They appear smaller than Loop I and were discovered in 2010 with about a year and a half of Fermi-LAT data. From observations of galaxies other than the Milky Way, we know of two possible mechanisms for creating such bubbles: emission from a supermassive black hole at the galactic centre, or a period of intensive star formation (a starburst) and supernova explosions. Which of these processes is responsible for the formation of the Fermi bubbles in our galaxy is not yet known.
Even the mechanism for producing the gamma rays in the first place is not yet resolved: it could be due to interactions between cosmic-ray protons and galactic gas, or inverse Compton scattering of high-energy electrons off interstellar radiation fields. Both of these options have caveats. For the first, it’s unclear, for instance, how one can collect and keep the high density of cosmic rays required to compensate for the low density of gas at large distances from the galactic plane. It’s also unclear whether the pressure of cosmic rays will expel the gas and create a cavity that will make the gas density even lower. For the inverse-Compton-scattering hypothesis, one would need electrons with energies up to 1 TeV. If these electrons were accelerated to such energies at the beginning of the expansion of the Fermi bubbles, then the bubbles’ expansion velocity would be about 10,000 km s–1 – at least 10 times larger than the typical observed outflow velocities.
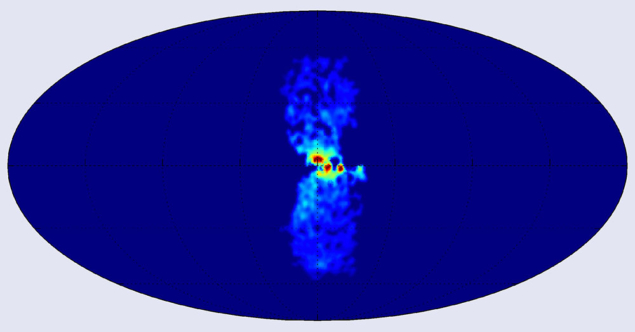
Moreover, even though the Fermi bubbles are similar in shape to gamma-ray lobes in other galaxies, which are typically visible in X-ray and radio wavelengths, they have no clear counterpart in X-rays and radio waves at high latitudes. Perhaps the Fermi bubbles are unique to the Milky way. Then again, perhaps astronomers have simply struggled to detect in other galaxies gamma-ray lobes that are “quiet” in the radio and X-ray bands.
A study of the gamma-ray emission from the Fermi bubbles at low latitudes could shed light on their origin, as it may point to the supermassive black hole at the galactic centre or to a region away from the centre, which would support the starburst scenario. Although the diffuse foreground and background gamma-ray emission from the Milky Way near the galactic centre is very bright, making it hard to interpret the observations, several analyses of Fermi-LAT gamma-ray data have revealed an increased intensity of gamma-ray emission from the Fermi bubbles near the galactic plane and a displacement of the emission relative to the galactic centre (figure 3). The higher intensity of the emission at the base of the Fermi bubbles opens up the possibility for a detection with ground-based very-high-energy gamma-ray Cherenkov telescopes, such as the upcoming Cherenkov Telescope Array, which is expected to start taking data with a partial array in 2022 and with the full array in 2025. At low energies, below 100 GeV, the flux from the base of the Fermi bubbles may also be confused with the third elephant in the sky – the galactic-centre GeV excess.
Galactic-centre GeV excess
The first hints of an extended excess of gamma rays from the centre and bulge of the galaxy at energies around a few GeV and with an almost spherical morphology were presented in 2009, before the discovery of the Fermi bubbles. However, given that the diffuse foreground gamma-ray emission along the galactic plane is very bright, and also rather uncertain towards the galactic centre, it took a long time to prove that the excess is not caused by mis-modelling of foreground components (such as inverse Compton scattering of high-energy electrons and hadronic interactions of the stationary distribution of cosmic rays along the line of sight). The spectrum of the excess has a peak at a few GeV, hence the name “GeV excess”, whereas the components of the diffuse foreground have a power-law structure around a few GeV.
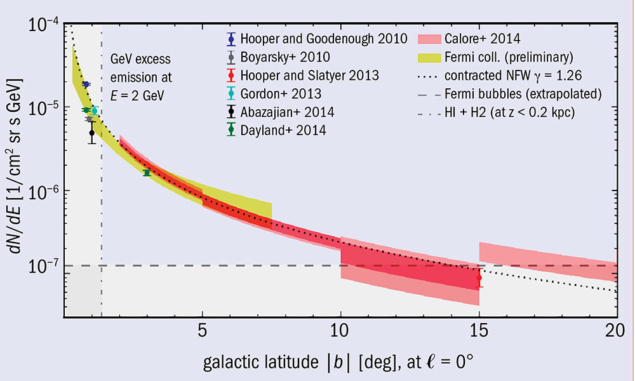
Intriguingly, the combined GeV centre and bulge emission has properties that are largely compatible with expectations from a dark-matter annihilation signal: the emission is extended, up to at least 10–15 degrees away from the galactic centre, with a profile that is consistent with that from a slightly contracted dark-matter-halo profile (figure 4). At energies below about 1 GeV, the gamma-ray emission grows steeply, and has a maximum at a few GeV with a cut-off or a significant softening at higher energies, which is expected for a signal from dark-matter annihilation.
Given the high stakes of claiming a discovery of a dark-matter annihilation signal, corroborating evidence for this hypothesis must be found, or alternative astrophysical explanations must be confidently excluded. Unfortunately, neither has happened up to now. Quite the contrary: there are several sources of gamma-ray emission near the galactic centre that could, within uncertainties, together account for all of the bulge and centre emission. For example, massive molecular-gas clouds near the galactic centre show clear indications of star-formation activity, which results in cosmic-ray production and associated gamma-ray emission in the inner galaxy. While the hadronic cosmic rays from such activity are not likely to explain the GeV excess, because their gamma-ray emission is not as extended as the GeV excess, inverse Compton emission from cosmic-ray electrons linked with such an activity can be extended over many degrees and is expected to contribute to the GeV emission. However, given that the energy spectrum expected for this inverse Compton emission is significantly flatter than the observed GeV excess, it is unlikely that this component accounts completely for the GeV-excess emission.
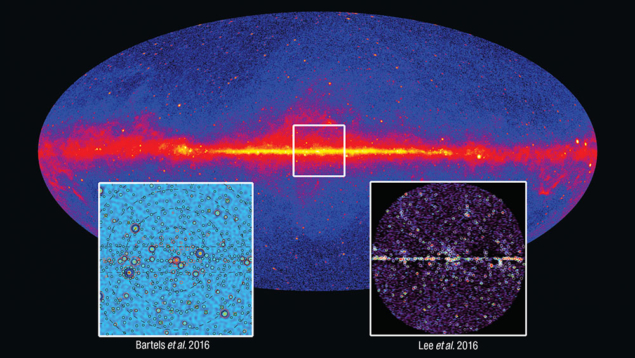
Arguably, the most plausible explanation for the GeV-excess emission from the galactic bulge and centre is a population of thousands of millisecond pulsars – highly magnetised neutron stars with a rotational period of 1–10 ms. They can emit gamma rays for billions of years before they lose energy, and their gamma-ray spectrum, as observed by Fermi-LAT, is similar to the spectrum of the GeV excess. It is plausible that millisecond pulsars in the bulge follow a similar spatial distribution as the majority of bulge stars. Indeed, recent analyses showed that the profile of the GeV-excess emission in the inner galaxy is better described by the boxy stellar bulge, rather than by a spherically symmetric dark-matter profile. Moreover, several detailed statistical analyses found evidence that the emission is more likely to be from a population of numerous but faint point sources, such as millisecond pulsars in the bulge, rather than from truly diffuse emission, such as that resulting from the annihilation of dark-matter particles (figure 5).
Future observations with radio telescopes such as MeerKAT in South Africa, expected to start taking data this year, and its successor, the Square Kilometre Array (SKA), the first construction phase of which is expected to end in 2020, should be able to test whether millisecond pulsars exist in the inner galaxy and can explain the GeV excess.
Additional multi-wavelength observations will provide new information about the three elephants in the sky. In particular, the eROSITA experiment, the successor of the X-ray ROSAT satellite, will survey the whole sky in X-rays and will be one order of magnitude more sensitive than ROSAT. With the eROSITA data, astronomers will search for a possible cavity carved out by cosmic rays in the Fermi bubbles and will estimate the distance to the North Polar Spur using the absorption of soft X-rays from the spur by the distribution of gas along the line of sight.
Possible connections
On the high-energy gamma-ray front, the upcoming Cherenkov Telescope Array is expected to detect the Fermi bubbles near the galactic plane above a few hundred GeV. This detection should help to answer the question of whether the Fermi bubbles are linked to the galaxy’s central supermassive black hole or to a different source away from the galactic centre. On the other side of the electromagnetic spectrum, the new generation of radio-telescope arrays, MeerKAT and SKA, should, as mentioned, be able to confirm or rule out the millisecond-pulsar hypothesis for the GeV excess. If the millisecond-pulsar hypothesis is excluded, then the dark-matter interpretation will remain as one of the plausible explanations. By contrast, a confirmation of the millisecond-pulsar hypothesis will significantly constrain the dark-matter hypothesis.
The presence of the three elephants in the gamma-ray sky in approximately the same direction raises the question of whether they are connected. One of the possible connections between the Fermi bubbles and Loop I is that Loop I is created by galactic gas pushed away by the expansion of the bubbles. In this case, the two elephants would become one, where Loop I is an outer part and the Fermi bubbles are an inner part. This scenario looks especially plausible for the northern bubble because Loop I extends beyond it.
The overlap between the GeV excess and the Fermi bubbles in the galactic-centre region provides the exciting possibility of a connection between the two. Models that try to explain the GeV excess with an additional population of cosmic-ray electrons, star formation and cosmic-ray acceleration processes, can connect the gamma-ray emission in the bulge with that at higher latitudes in the Fermi bubbles. Also, the mechanism underpinning the formation of the bubbles – whether it is linked to activity of the galaxy’s central supermassive black hole or to a burst of star formation – might affect the properties of the GeV excess. Future observations and analyses will help to settle the nature – common or not – of the three elephants in the sky, and might point to new physics such as dark-matter annihilation in the Milky Way. Studying the gamma-ray sky will no doubt be an exciting journey for many years to come.
Further reading
R Bartels et al. 2016 Phys. Rev. Lett. 116 051102.
F Calore et al. 2015 Phys. Rev. D 91 063003.
The Fermi-LAT Collaboration 2017 Astrophys. J. 840 43.
S K Lee et al. 2016 Phys. Rev. Lett. 116 051103.
D Malyshev (ed) 2018 Searching for Connections among the Fermi Bubbles, the Galactic Center GeV Excess, and Loop I Galaxies (special issue)
M Remazeilles et al. 2015 Mon. Not. R. Astron. Soc. 451 4311.