A seminal meeting in 1976 shaped the course of neutrino detectors.
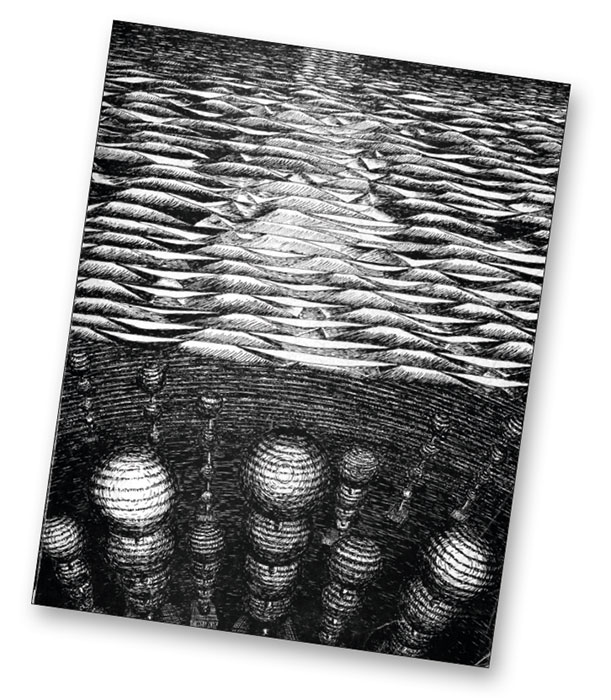
Image credit: Rene Donaldson, Fermilab.
In 1976, neutrinos did not yet have the prominent role in particle physics that they play today. Postulated by Pauli in 1930, they had been said to be undetectable due to their tiny interaction probability, and were only first observed in the mid-1950s by Fred Reines and Clyde Cowan using a detector located close to a military nuclear reactor. In 1962, researchers at Brookhaven National Laboratory discovered a second type of neutrino, the muon neutrino, but the third (tau) neutrino would not be seen directly for a further 38 years. On the other hand, the theory of electroweak interactions mediated by the W and Z bosons was firming up, and measurements of neutrinos played a significant role in this context.
The first naturally generated neutrinos, originating from cosmic-ray collisions in the Earth’s atmosphere, were observed in 1965 in deep gold mines located in South Africa and India. Also in the late 1960s, Ray Davis was beginning his famous solar-neutrino observations. The time was right to start thinking seriously about neutrino astronomy.
Enter DUMAND
Plans that ultimately would shape the present-day neutrino industry blossomed in September 1976 at a meeting in Waikiki, Hawaii. It was here that some of the first ideas for large detectors such as the gigaton DUMAND (Deep Underwater Muon and Neutrino Detector) array, which eventually morphed into the present-day IceCube experiment at the South Pole, were envisioned. The technology for smaller water-filled detectors such as IMB (Irvine–Michigan–Brookhaven) and, later, Kamiokande in Japan, were also laid out in detail for the first time. Moreover, totally new concepts such as particle detection via sound waves or radio waves were explored.
The first organised stirrings of what was to become DUMAND took place at a cosmic-ray conference in Denver, Colorado, in 1973, which led to a preliminary workshop at Western Washington University in 1975. It was at this meeting that the detection of Cherenkov radiation in water was chosen as the most viable method to “see” neutrino interactions in a transparent medium, with some deep lakes and the ocean near Hawaii considered to be good locations. This detection principle goes back to Russian physicists Moisey Markov and Igor Zheleznykh in 1960: water provides the target for neutrinos, which create charged particles that generate a flash of light in approximate proportion to the neutrino energy. The water also shields the many downward-moving muons from cosmic-ray interactions in the atmosphere. With light detectors, such as a basketball-sized photomultiplier tube, one could register the light flash over large distances.
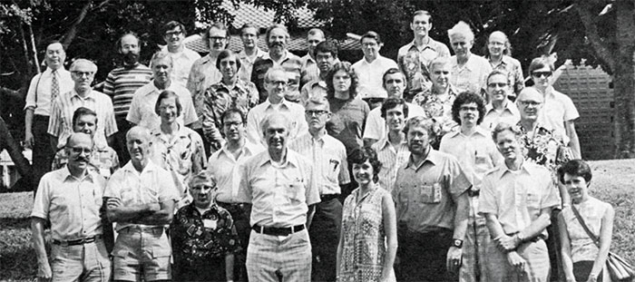
Image credit: Anatoly Pethruhkin.
The vision of building devices larger than any yet dreamed about, and placing them in the deep ocean to study the cosmos above, attracted many adventurous souls from around the US and elsewhere, a number of whom dedicated years to realising this dream. One of us (JL) joined Reines, along with Howard Blood of the US navy and other ocean-engineering aficionados – in particular, George Wilkins, who was responsible for the first undersea fibre-optic cables. Inventor of the wetsuit and former bubble-chamber developer Hugh Bradner became another driving force behind the project. Theorists, cosmic-ray experts, particle physicists, astronomers and astrophysicists all played important roles (see photograph). The event captured a spirit of adventure and worldwide co-operation, particularly concerning interactions between US and Soviet colleagues, and the special nature of our unusual international physics collaboration brought a certain level of spice to relations.
Searching the sky
A significant problem of the era was to know what sources of neutrinos the detectors should be looking for. Astrophysicists and astronomers seldom thought about neutrinos at the time, and neutrinos were neglected in calculations of radiation and power in the universe. They were, however, included in studies of solar burning and supernovae, and it was from these efforts that neutrinos began to appear in the astronomer’s lexicon. For the first time, a survey of possible astrophysical sources of lower-energy neutrinos (typically 1–100 MeV) was produced at the 1976 meeting, which were organised by Craig Wheeler into seven possible steady sources and eight potential burst sources.
For the steady sources, only solar neutrinos and terrestrial radioactivity appeared practical for detecting neutrinos. For the bursting neutrino sources, galactic gravitational collapses clearly yielded the most available total power for neutrinos. The others – namely type I supernovae, solar flares, gamma- and X-ray bursts and mini-black-hole evaporation – did not seem to have enough power and proximity to be competitive. Even today, these sources have not been observed in terms of neutrinos.
There was also great interest in targeting high-energy (TeV and above) neutrinos, but predictions of the strengths of the potential sources were plagued by huge uncertainties. It was known that the number of cosmic rays impinging upon the Earth decreases as a function of energy up to values of around 1020 eV, whereupon the spectrum was predicted to show a cut-off known as the Greisen–Zatsepin–Kuzmin (GZK) limit caused by high-energy protons being degraded by resonant collisions with the cosmic microwave background. Veniamin Berezinsky of the Lebedev Institute in Moscow put forward some prescient models of ultra-high-energy neutrino generation, in particular, those generated in GZK processes, and also gave first estimates of upper bounds on neutrinos from star formation in the early universe. Credible sources of neutrinos with energies far beyond the TeV scale were probably too far ahead of people’s visions at the time, while neutrino cross-sections and even the production dynamics were not well defined at the highest energies.
By the end of the Hawaii workshop, which lasted for two weeks, everyone considered low-energy neutrino detection to be the most worthwhile cosmic-neutrino-detection goal. Aside from solar neutrinos, it was also agreed that neutrinos from supernova collapses were the most likely to be seen (as they later were in 1987, when a burst of neutrinos was observed by the IMB, Kamiokande and Baksan detectors). But it was also realised that this effort requires kiloton detectors with threshold sensitivities of about 10 MeV to guarantee a few supernovae per century. Regarding the detection of high-energy neutrinos in the TeV range, as expected from acceleration processes in galactic and extragalactic objects, everyone understood the necessity of detectors in the megaton to gigaton class. This was so far beyond the reach of technology at the time, however, that people realised they had to start small and work upwards in target mass.
Interestingly, neutrinos generated in the atmosphere with energies in the GeV range were regarded as the least interesting target. Nobody at that time would have expected that precisely these neutrinos, together with solar neutrinos, would demonstrate for neutrino oscillations and lead to the award of the 2015 Nobel Prize in Physics.
Detector evolution
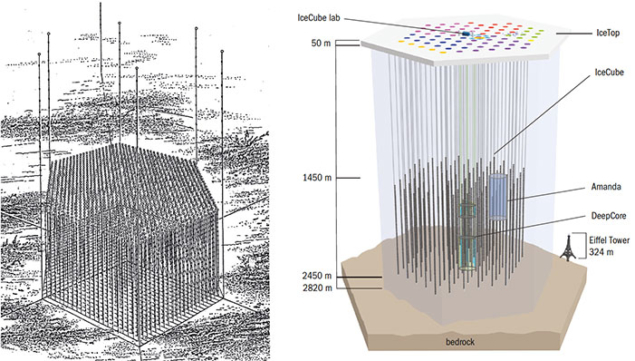
Image credit, right image: IceCube.
Considering the vast target volumes required for high-energy neutrino astronomy, it became clear that the most promising – and most affordable – detection method was to register the Cherenkov light in natural water. With the intensity of Cherenkov light being around 30 times weaker than that from a scintillator, however, the design of the optical detectors became paramount. One group of attendees from the 1976 workshop aimed for the use of wavelength shifters that absorb blue light and re-emit green light, allowing the use of modest photodetectors, while another pushed for the development of photomultipliers larger than the 25 cm-diameter versions available at the time – as did in fact transpire. The one serious alternative to optical Cherenkov light detection, building on a concept developed by Gurgen Askaryan in 1957, was to utilise the pulse of sound made by neutrino progeny after neutrino collision with a nucleus of water or another medium such as ice.
Following the 1976 event, annual neutrino workshops were held for about a decade, eventually blending with DUMAND collaboration meetings. The 1978 workshop, held in La Jolla, California, took place in three sessions over a six-week period, and attracted more neutrino converts from physics, astrophysics and ocean engineering. The following year’s event, which was held at Khabarovsk and Lake Baikal in Siberia, offered some physicists their first chance to interact with Soviet physicists who had not been able to travel. Indeed, by the end of 1979, international politics and in particular the Soviet–Afghan war had forced the separation of the Russian and US DUMAND efforts. Russian DUMANDers decided to push ahead with a detector array deployed from ice in the world’s deepest freshwater lake, Lake Baikal, and one of us (CS) joined the Baikal collaboration in 1988. A few years later, the first underwater neutrino events were identified in Lake Baikal, and the principle of this detection technique was finally proven (followed by more statistics from AMANDA at the South Pole and ANTARES in the Mediterranean Sea). The heroic efforts of Russian physicists through difficult times have continued for 35 years, and Baikal researchers have just deployed the first subunit of a cubic-kilometre array similar to IceCube.
The formal outcome of the 1976 workshop was a joint resolution and plans for ocean studies and further workshops. The major vision for the high-energy DUMAND detector itself (see diagram) was an array of bottom-moored strings carrying some 22,000 optical detectors distributed in a volume slightly larger than one cubic kilometre – quite similar to the eventual IceCube array. The DUMAND project carried out many ocean studies and also accomplished some physics offshore in Hawaii, measuring muons and the lack of large bursts.
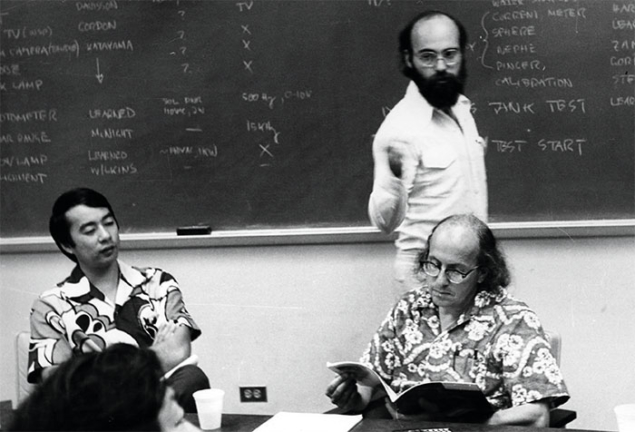
Image credit: Anatoly Pethruhkin.
Alas, DUMAND was cancelled by the US Department of Energy in 1995, prior to starting full deployment. One can debate the causes – the failure of the first deployed string was certainly one aspect. It may be noted, however, that the Superconducting Supercollider had just been cancelled and that the main funding agencies were simply not supportive of non-accelerator research until after SuperKamiokande’s discovery of neutrino oscillations in 1998. The DUMAND project was also ahead of its time in terms of its detection scheme, the use of undersea fibre optics and robotic module deployment.
The DUMAND legacy
Attendees of DUMAND’76 realised the importance of the venture, but probably came away with varying levels of belief in its practicality. Perhaps the greatest legacy of the 1976 workshop was bringing natural neutrino studies to the attention of a wide research community, and astrophysicists in particular. The breadth of ideas that this allowed, and the spirit of interdisciplinarity and international co-operation, has continued in the neutrino community. Moreover, initially still-born alternatives for neutrino detection have been revived in the last two decades.
The neutrino-detection method using acoustic signals was taken up in the late 1990s and early 2000s using military hydrophone arrays close to the Bahamas and to Kamchatka, as well as dedicated test set-ups in Lake Baikal, the Mediterranean Sea and in Antarctic ice – namely, the South Pole Acoustic Test Setup (SPATS). More profitably, the radio technique of detecting high-energy neutrino interactions via the negative charge excess in a very-high-energy particle shower induced by a neutrino interaction has allowed stringent upper limits to be placed on neutrino fluxes at the highest energies. The Askaryan Radio Array (ARA), the balloon-borne ANITA project and ARIANNA all focus on radio detection in Antarctic ice. Indeed, ANITA has just reported the first candidate for a super-high-energy neutrino event emerging from the Earth at a large angle.
Probably the most important lineage descending from the 1976 workshop is in the exploitation of the large water Cherenkov detectors located underground: IMB (1983), Kamiokande (1985) and, most notably, SuperKamiokande (1996). Some other experiments can be claimed as at least partial progeny of the early DUMAND enterprises, such as NESTOR in the Mediterranean, the Sudbury Neutrino Observatory (SNO) in Canada and KamLAND in Japan. The links become more tenuous, but the people engaged all owe much stimulation and experience to the explorations of these problems and detector solutions 40 years ago.
As for DUMAND itself, its spirit lives on in present-day neutrino observatories. IceCube at the South Pole is certainly the most extreme realisation of the DUMAND concept, and DUMAND spherical phototube modules are still the archetypal unit for this and many other detectors, such as ANTARES and GVD in Lake Baikal. A further array currently being installed deep in the Mediterranean, KM3NeT, has varied the principle by arranging many small phototubes inside of the glass sphere instead of a single large tube, while IceCube is exploring multiphototube modules as well as wavelength-shifter solutions for its next-generation incarnation.
Following IceCube’s discovery of the first extraterrestrial high-energy neutrinos in 2013, we are finally realising the decades-old dream of seeing the universe in neutrinos. Today, with thousands of researchers undertaking neutrino studies, the revelations for particle physics from neutrinos seem to be unending. The experimental road has been full of surprises, and neutrino physics and astronomy remain some of the most exciting games in town.