A new range of PET instrumentation, based on research and development experience from high-energy physics experiments, aims to provide biology researchers with powerful tools to monitor the effects of gene therapy and to screen and test the effects of new drugs.
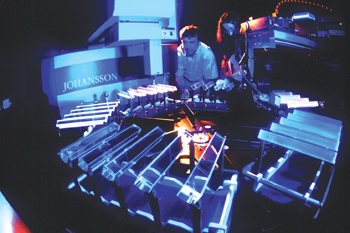
At the front line of medical research, molecular and cellular biologists engineer new molecular probes, including genes and proteins. Having produced them, the next task is to investigate what happens when they are inserted into living tissue.
In biology-speak, the researchers want to know how and where the new genes “express” themselves. In pharmaceutical research, the effects of potential new drugs have to be established as quickly and as cost effectively as possible.
In the past, results have been established in vitro either by sacrificing the animal and analysing samples, or by taking biopsies. Until recently there has been no easy way of studying the effects of genetic manipulation or drug administration in living animals and in real time.
Now researchers have found how imaging techniques used in medical diagnosis can be adapted for genetic or drug research, providing an immediate picture of how the modified tissue behaves while it is still alive – in vivo and in situ. The techniques being used are magnetic resonance imaging (MRI), and positron emission tomography (PET).
Creating new images
In 1952 the Nobel Prize for Physics was awarded to Felix Bloch, who in 1954 became CERN’s first director general, and Edward Purcell for their discovery of nuclear magnetic resonance (NMR). In this technique, nuclei gripped by a strong magnetic field are exposed to microwave radiation.
NMR was used initially with uniform magnetic fields to study molecular structure. More than 25 years later, researchers began to realize how the use of non-uniform magnetic fields could produce position-dependent signals, from which a computer could reconstruct a three-dimensional structural image. The time resolution of MRI was soon improved, so that, for example, heart function could be monitored.
The other technique, PET, works by administering harmless but selective radioisotopes that emit positrons – the antiparticles of normal atomic electrons. These isotopes are taken up by molecules involved in the metabolic functions of cells or organs and they work their way into the part of the organism being studied (such as the brain). Eventually the emitted positrons annihilate with atomic electrons, each annihilation producing a characteristic back-to-back pair of 511 keV photons (gamma rays).
Monitoring the distribution of these gammas reveals the detailed structure of where the isotope becomes localized. For instance, cancer cells are known to have a more rapid metabolism than normal cells, consuming more energy in the form of glucose. After injection, a fluorine-18 positron emitter in the form of fluorodesoxyglucose molecules (FDG) is taken up by cancer cells and accurately reveals primary cancers and metastasic activity. PET also provides a “moving image” of the metabolic function of the tissue or organ. This is particularly useful for genetic and drug research, showing how the organism is being affected as well as where.

Since its inception, PET technology has continually benefited from new developments in radiation detection, first using sodium iodide crystals, then benefiting from the improved performance from bismuth germanate (BGO) and, more recently, exploiting superior materials such as lutetium orthosilicate or aluminate, which are faster and more effective than BGO.
Monitoring gamma rays is a vital task in any major physics detector, particularly for the component studying the absorption of energy via electromagnetic processes – the electromagnetic calorimeter. In this module of the detector, tiny scintillations of light produced by electromagnetic interactions are collected, amplified if necessary and analysed.
As part of the preparations for the experimental programme for CERN’s Large Hadron Collider (LHC), the Crystal Clear research and development collaboration was established in 1990 by Paul Lecoq from CERN to look into the development of new scintillating materials.
After input from different disciplines (crystallography and solid-state physics, as well as particle physics), early accomplishments led to the decision by the collaboration for the CMS experiment at the LHC to use lead tungstate (PbWO4) for its electromagnetic calorimeter. The production of the 80,000 crystals for CMS is the subject of a major international collaboration.
In parallel, the collaboration, now led by Stefaan Tavernier from the Vrije University in Brussels, established the use of cerium fluoride (CeF3) as another high-energy physics standard, and worked with specialist companies in the US, China and the Czech Republic to ensure its production on the industrial scale. Heavy scintillating fluoride glasses is another growth area, where the collaboration works with a French company. The Crystal Clear collaboration also pioneered the use of new compounds based on lutetium.
Working with the Czech Crytur company (also involved in work for cerium fluoride), the collaboration has developed scintillating crystals of yttrium aluminate perovskite (YAP) doped with increasing amounts of lutetium, which gives twice as much light as BGO and over a broader range of wavelengths. YAP is extensively used in medical instrumentation and in screens for electron microscopes but its density is marginal for high sensitivity PET applications that have to stop 511 keV photons. Replacing up to 100% of yttrium by lutetium ions gives a very bright and fast scintillator, LuAP, with the unprecedented density of 8.34g/cm3.
Smaller instrumentation
Physics detectors are normally large – even a small one is several metres long. However, PET cameras for medical use are more compact. Even so, the spatial resolution of commercially available cameras meant that PET analysis was, until recently, limited to relatively large subjects, such as humans and other large animals. New PET techniques extend this approach to smaller specimens, and this is where the technique becomes interesting for genetic laboratory studies.
In the Crystal Clear collaboration, YAP, lead tungstate and lutetium aluminate are all being investigated for their possible use in small PET scanners. On the read-out side, additional amplification can complement the traditional photomultiplier read-out of scintillation light. This can be achieved by avalanche photodetectors using applied bias voltages or by imaging silicon pixel array (ISPA) tubes using internal photocathodes and semiconductor read-out. Italian groups have achieved submillimetre resolution using YAP-based cameras.
New PET scanners (ClearPET) for biological research are the subject of collaboration agreements between the Crystal Clear collaboration and specialist local centres in France, Belgium, Switzerland and Germany. A project for a mammography PET camera (ClearPEM) is under discussion with Portugal.
The work and achievements of the Crystal Clear collaboration are a good example of how particle physics expertise can seed important developments in other areas of science. Rather than being left to happen fortuitously, such symbiosis is now being encouraged and nurtured at CERN.