Michael Albrow’s personal walk through the legacy of the ISR.

It is difficult to imagine a greater contrast than that between the particle detectors installed at the ISR, when the first proton–proton collisions took place 40 years ago, and those ready for the first collisions at the LHC in 2009. Several experiments were waiting in the wings, but in January 1971 just a few simple scintillation counters were in place to detect the first collisions at the ISR, while an oscilloscope trace showed left-moving and right-moving beam halo and some left–right coincidence signals from collisions.
The ISR was in many ways a “transitional machine”, a bridge between relatively low-energy, fixed-target accelerators and today’s extremely high-energy colliders, as well as between detectors based largely on scintillation and Cherenkov counters, spark chambers or bubble chambers and today’s (almost) full-solid-angle trackers, calorimeters and muon detectors that record gigabytes of data per second. For example, the last large ISR experiment, the Axial Field Spectrometer (AFS), pictured right, with its full-azimuth drift chamber and uranium-scintillator calorimeter, bore no resemblance to any of the first-generation experiments but had much in common with the detectors found in later colliders. Also, from the theoretical point of view, the decade of the ISR saw the transition from confusion to today’s Standard Model, even though other machines made some dramatic key discoveries – charm, the W and Z bosons, and the third family of quarks and leptons.

Before the start of the ISR, the idea that fractionally charged quarks could be produced there led to a special session of the ISR Committee (ISRC-70-34) that reviewed eight quark-search proposals, of which three were “encouraged”. It was later established that fewer than one charged particle in 1010 has a charge 1/3 or 2/3. It would be a stretch to claim that this was “observation of quark confinement”, but being at a higher energy than other accelerator experiments and with much greater sensitivity than cosmic-ray studies, the ISR played a role in our current belief that free quarks do not exist outside hadronic matter. However, quarks can still be “seen” confined inside hadrons – as the deep-inelastic, electron-scattering experiments at SLAC discovered in 1968.
In 1971, today’s theory of strong interactions, QCD, was also “waiting in the wings”; theorists were groping towards the light. Simple (experimentally, not theoretically) two-body reactions such as proton–proton (pp) elastic scattering or π– + p → π0 + n were described by Regge theory, which was based on the sound principles of unitarity (no probabilities higher than 1.0), analyticity (no instantaneous changes) and the crossing symmetry (“what goes in can come out”) of scattering amplitudes. While Regge theory is still a more useful approach than QCD for those reactions, calculations became difficult because the strong interaction between hadrons is strong and the calculations do not converge. It was also clear that at the higher ISR collision energies – jumping from the 28 GeV beams of the Proton Synchrotron (PS) at CERN and the Alternating Gradient Synchrotron (AGS) at Brookhaven to an equivalent beam energy of 2000 GeV – many hadrons could be created and that Regge theory had little to say about it except for certain “inclusive” reactions, discussed below.
At the first ISRC meetings in 1968 and 1969 the decision was taken to devote one of the eight intersection regions to a “large, general-purpose magnet system”. Three systems had been proposed and a working group was asked to make a rapid decision. The choice fell on the Split Field Magnet (SFM) – primarily because its field was strong and simple (a dipole) in the forward directions, where most particles would be produced. Unfortunately, the field was zero at 90° and, with pole pieces above and below the beams, it was unsuitable for physics at high-transverse momentum, pT. By 1978 the SFM had been upgraded with greatly improved detectors, but it remained focused on forward and diffractive particle production.
Hadronic diffraction at high energies, the simplest example being elastic scattering, is described in Regge theory as arising mainly from the exchange of a pomeron between the scattering protons. This has quite different properties from other, virtual meson (or “Reggeon”) exchanges. Before the ISR, the total pp cross-section was known to decrease with energy, as it did for πp (but not for K+p). The early discovery that it rises (as in figure 1), which was a surprise to many, had been predicted if, and only if, the pomeron is an allowed exchange. Today we take it for granted that the total pp cross-section rises with energy but at the time the rise led to much experimental and theoretical activity: does the proton become more opaque? Or larger? Or both? Beautiful experiments, for example by the CERN-Rome group that developed “Roman pots” to place detectors very close to the circulating beam, showed that the slope (in momentum transfer, t) of elastic scattering increases with energy. Thus protons in effect become larger but they also become more opaque. Roman pots have been used at all subsequent hadron colliders, including the LHC.

The first ISR experiments were mostly concerned with strong interactions at large distances, or small momentum transfers. On the menu, in addition to searches for free quarks, monopoles and weak vector-bosons, were elastic scattering and low- and high- multiplicity final states. How could such complicated final states be handled experimentally? A popular approach, still common today, was to measure the angular and momentum distributions of a single particle from each collision and ignore all of the others – the so-called “inclusive single particle” spectra. As mentioned, Regge theory could be adapted to describe such data, but only at low pT. Experiment R101 (intersection 1, experiment 1) was simplicity itself: literally a toy train with photographic emulsions in each wagon. When colliding beams were established it was shunted alongside the collision region, and left there to measure the angular distribution of produced particles. The first physics publication from the LHC was of the same distribution, although not measured with a toy train set!
Pre-ISR experiments at the PS typically installed detectors for a few weeks or months and then moved on. It was (jokingly?) said that you should not have more photomultipliers than physicists. That mindset persisted in the early ISR days. Four experiments shared Intersection 2 (I2). Three single-arm spectrometers measured inclusive particle spectra at small and large angles. They discovered Feynman scaling – in which forward particle spectra are proportional to the beam energy – at small (but not at large) pT, high-mass diffraction and co-discovered high pT particles. Feynman scaling was shown to be approximate only; indeed, scaling violations are a key feature of QCD. Two of these spectrometers were combined in 1975 to look for hadrons with open charm but, in retrospect, the acceptance was far too small. The fourth experiment at I2 was a large, steel-plate spark chamber designed to look for muons from the decay of the then-hypothetical W boson, supposing its mass might be only a few giga-electron-volts. (It was later found to have a mass of 81 GeV, much too high for the ISR.) Unfortunately, with hindsight, the muon detector was not made in two halves on opposite sides so as to have more acceptance for muon pairs; had the collaboration persevered as the luminosity increased they might have seen J/ψ → μ+μ–. One reason they gave for not persisting was that the background from charged π → μ decays was much larger than they had expected.
The reputation of the ISR as a physics-discovery machine suffered greatly from missing the discovery of the J/ψ particle, which made its dramatic entrance in November 1974 at Brookhaven’s AGS and the e+e– collider at SLAC. The “November Revolution” convinced remaining doubters of the reality of quarks, with important implications for electroweak interactions. How did the ISR miss it? There is no single answer. Today’s intense interaction between theorists and experimenters hardly existed in the early 1970s – but even if it had, there would have been few, if any, voices insisting on a search for narrow states in lepton pairs.
R103, one of the early experiments designed to measure electron (and π0) pairs by the CERN-Columbia-Rockefeller collaboration (CCR), already had two large lead-glass arrays on opposite sides of the collision region in 1972–1973 and found an unexpectedly high rate of events. This was the important discovery of high-pT hadron production from quark and gluon scattering, but it had the unfortunate consequence that the team had to turn their trigger threshold (with 10 Hz rate-limited spark chambers) up to 1.5 GeV, just too high to accept J/ψ → e+e–. This was followed in 1974 by R105 (by CCR plus Saclay), which included a gas Cherenkov counter. There were about a dozen J/ψ events on tape at the end of 1974 but not clear enough and not in time for a discovery. However, before November 1974, R105 (together with Fermilab experiments) had already discovered direct lepton production, in a proportion e/π of 10–4, which was later described by a “cocktail” of processes (J/ψ, open charm and Drell-Yan qq annihilation).
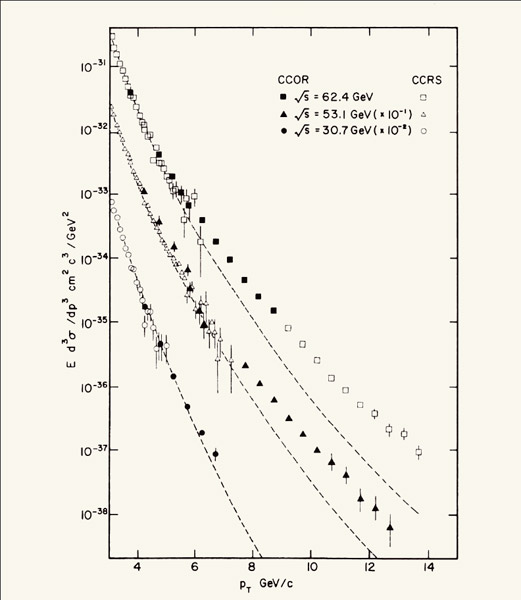
High-pT particle production was not promoted by theorists until after the ISR started up. In December 1971, Sam Berman, James Bjorken and John Kogut (BBK) used Richard Feynman’s parton model, which was supported by deep-inelastic electron scattering, to predict a much higher production rate of hadrons and photons at high pT than expected from a simple extrapolation of the then-known exponentially falling spectra (Berman et al. 1971). The rates that they calculated were for electromagnetic scattering of the charged partons, but they noted that these were lower bounds and strong scattering (the exchange of a spin-1 gluon) would give much larger cross sections. They also suggested that scattered partons (now known to be quarks and gluons) would fragment into jets (“cores”) of hadrons along the direction of the parent parton. Feynman had similar ideas.
The observation of unexpectedly high rates of high-pT hadron production at the ISR was a major discovery (figure 3); it showed that parton–parton scattering indeed occurred through the strong interaction, but with a weaker coupling than between two protons. This behaviour was later understood in QCD in terms of a decreasing strong coupling at smaller distances – the phenomenon of asymptotic freedom for which David Gross, David Politzer and Frank Wilczek received the Nobel Prize in Physics in 2004. Unfortunately, the high pT discovery – made by the CCR collaboration (for π0) and the British–Scandinavian and Saclay-Strasbourg collaborations (for charged hadrons) – masked the J/ψ in the e+e– channel. As noted earlier, high-pT pions produced an unexpected large background also to muon measurements, so the muon pairs were not pursued.
The high-pT jets predicted by BBK took another decade to be discovered in hadron–hadron collisions, almost 10 years after jets had been seen in e+e– collisions. One needed to select events with large, total transverse energy in an area much greater than the jets themselves, and with a hadron calorimeter with excellent energy resolution as in the AFS (see photo p39). After a long struggle, the collaborations of the AFS (R807) at the ISR and UA2 at the Super Proton Synchrotron (SPS) running in proton–antiproton (pp) collider mode (SppS), submitted papers on the same day to Physics Letters with convincing evidence for jets. The ISR data extended to a jet-transverse energy, ET = 14 GeV, but the SppS data reached 50 GeV with 1/1000 the luminosity of the ISR. At all post-ISR colliders, high-ET jets are considered as “objects” that are almost as clear as electrons, muons and photons. The experiments at the LHC are already studying the 2-jet mass spectrum for evidence of new particles with masses of up to 2 TeV.
The scattering of two quarks is described in QCD by the exchange of a gluon – the strong-force equivalent of the photon. Gluons must also be present as constituents of protons, being continuously emitted and absorbed by quarks. The “discovery of the gluon” is credited to the observation at DESY of e+e– annihilation to three jets, which showed clearly that outgoing q and q jets could be accompanied by gluon radiation. Although not as dramatic, it was clear at the ISR that the high-pT particle production required more scattering partons in the proton than just the three valence quarks, and that the inclusion of gluons gave sensible fits to the data.
A related ISR discovery was the production of high-pT photons, produced directly rather than coming from the decay of hadrons (such as π0); these are direct probes of the processes q + q → g + γ and q + g → q + γ. Direct high-pT γγ production was later observed. Now, at the LHC, direct γγ production is a promising search channel for the Higgs boson.
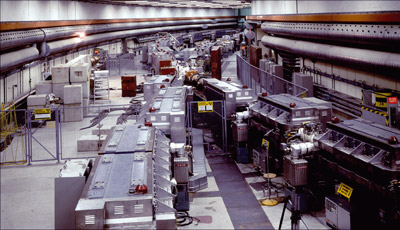
With the advent of the parton model most physicists – theorists and experimenters – were happy to be able to leave the complicated, difficult world of hadrons at the femtometre scale and dive down to the next, partonic, layer, which was both simpler theoretically and experimentally exciting. But what they left behind is still unfinished business. While QCD is frequently said to be the theory of strong interactions it still can not calculate hadron processes. Every hadronic collision involves large-distance processes, which we are not yet able to calculate using QCD. The problem is that the strong interaction becomes too strong when distances become as large as the size of hadrons (about 1 fm); indeed, this is responsible for the permanent confinement of quarks and gluons inside hadrons. Calculations that work well on smaller distance scales (or larger momentum transfers), do not converge; they blow up and become intractable.
So, while QCD cannot be used to calculate small-angle elastic scattering, Regge theory with pomeron exchange can describe it, although we recognize that it is less fundamental. High-mass diffraction provided a new tool for studying pomeron exchange and eventually double-pomeron reactions such as pomeron + pomeron → π+π– (and to other hadron states) were found. We now understand that the pomeron is, to leading order, a colourless pair of gluons. The idea that there could be quarkless hadrons, or “glueballs”, also motivated these studies. Not finding them implied that if they exist they must be heavy (at least about 1 GeV) and so short-lived that they could not emerge from the collision as free hadrons.
The pomeron itself is not a particle, but an exchanged “entity” with Regge properties (complex angular momentum, negative mass-squared). Heroic attempts have been made to calculate its properties in QCD. Perhaps one day Regge theory will be proved to be a large-distance limit of QCD. While at the ISR, central masses in double-pomeron reactions were limited to less than about 3 GeV, at the LHC they extend to masses a hundred times greater, allowing Higgs bosons – if they exist – to be produced in the simple, final state p + H + p, with no other particles produced. Both the ATLAS and CMS collaborations have groups proposing to search for this process, which can be called “diffractive excitation of the vacuum” because the Higgs field fills (in some sense “is”) the vacuum.
A string theory of hadrons was briefly in vogue in the 1970s, with qq mesons as open strings and pomerons as closed strings. Regge theory is compatible with this idea and can explain the relationship between the mass and spin of mesons. Thirty years later, string theory is in vogue once again but on a much smaller, near-Planck scale, with electrons and quarks as open strings and gravitons being closed strings. Despite the enormous progress in collider technology, no one can imagine a collider that could see such superstrings, unless extra dimensions exist on an LHC scale.
Many other studies of strong interaction physics were made at the ISR. These included particle correlations, short-range order in rapidity, resonance production etc. Multiparticle forward spectrometers also made systematic studies of diffraction, including the production of charmed baryons and mesons.
With its two independent rings, the ISR was more versatile than any other collider – then or since. Not only were pp collisions studied, but antiprotons and deuterons and α-particles were also made to collide with each other and with protons. For the last run, an antiproton beam was stored in the ISR for more than 350 hours, colliding with a hydrogen gas-jet target to form charmonium. So the swansong of the ISR was a fixed-target experiment measuring the very particle that it had missed because high pT physics got in the way!
The ISR machine was outstanding and the detectors eventually caught up and led the way to the modern collider physics programme. When it was closed in 1984, there was still plenty to do, despite the higher energy SppS collider, whose UA1 and UA2 detectors owed so much to the ISR experience. However, the ISR had to make way for the Large Electron–Positron collider, which in turn made way for the LHC, so that proton–proton collisions are once again exciting.
• This has been a personal, and far from comprehensive, view of the physics that we learnt at the ISR. I thank Leslie Camilleri, Luigi Di Lella and Norman McCubbin for careful reading and redressing some balance. I also pay homage to Maurice Jacob, who did so much to bridge the gap between theorists and experimenters.