Chan Joshi reviews recent progress with plasma-based accelerators.

Image credit: F Tsung, UCLA.
Experiments at accelerators have produced many key breakthroughs in particle physics during the past 50 years. Today, as exploration begins of physics at the “terascale”, the machines needed are extremely large, costly and time-consuming to build. In 1982, however, recognizing that this is how the field would evolve, the US Department of Energy (DOE) began a programme to develop new ideas for particle acceleration, which has now become extremely active. From the outset it was clear that developing an entirely new concept for accelerating charged particles would be a multi-disciplinary endeavour, requiring a sustained research effort of several decades to come to fruition (HEPAP 1980). Here I would like to examine just how far one advanced concept – plasma-based particle accelerators – has come after 20 or so years of research, and to indicate how it is likely to develop in the next decade.
Historical background
The first suggestions for using “collective fields” generated by a medium-energy electron beam to accelerate ions to high energies can be traced to Gersh Budker and Vladimir Veksler. However, plasma-based accelerators did not take off until John Dawson and his co-workers at the University of California, Los Angeles (UCLA) proposed the use of a space-charge disturbance, or a “wakefield”, to accelerate electrons (Joshi 2006; Joshi and Katsouleas 2003). Serendipitously, the ideas that Dawson developed between 1978 and 1985 coincided with the DOE’s initiative on advanced accelerator techniques and were supported first in the US and then in other countries.

Wakefields in a plasma can be driven by an intense laser pulse (the laser-wakefield accelerator) or an electron-beam pulse (the plasma-wakefield accelerator) that is about half a plasma wavelength long. In the former it is the radiation pressure of the laser pulse that pushes away the plasma electrons, whereas in the latter this is achieved by the space-charge force of the (highly relativistic and therefore stiff) electron beam. The plasma electrons are predominantly blown out radially, but because of the space-charge attraction of the plasma ions, they are attracted back towards the rear of the laser (or the particle) beam where they overshoot the beam axis and set up a wakefield oscillation. In a 1D picture the wake resembles a series of capacitors where the mostly transverse electric field of the laser (particle) beam has been transformed into a longitudinal electric field of the wake. Charged particles in an appropriately phased trailing pulse can then extract energy from the wakefield (figure 1a).
The mixture of physics disciplines involved meant that even proof-of-concept experiments on plasma accelerators required an expertise in plasma physics, lasers and beam physics. Since such expertise resided in universities, most of the early work was carried out by small university groups. By the 1990s many teams around the world had confirmed that plasma wakes did indeed have accelerating gradients of the order of 100 GeV/m and could accelerate electrons, often trapped from the plasma itself, with a continuous energy spectrum up to 100 MeV. However, there remained two important goals for the proponents of plasma-based accelerators to provide beams of interest to the end user of this technology – the high-energy physics community. They needed to show that plasma accelerators could produce a “monoenergetic beam” of electrons and that the high-gradient acceleration could be maintained over scales of a metre. There has been significant progress in achieving both of these goals in the past couple of years.
The “plasma bubble” accelerator
Most laser-driven and particle-driven plasma-wakefield accelerators now operate in the “bubble regime”. Here the drive pulse is so intense that it expels all of the plasma electrons for which subsequent trajectories enclose a “bubble” of ions. The resulting wakefield structure is 3D and the longitudinal wakefield is highly nonlinear (figure 1b). The phase velocity of the wakefield is tied to the group velocity of the drive beam, which is approximately the velocity of light, c.
In present laser-wakefield accelerator experiments, even though the phase velocity is relativistic, the accelerating particles eventually outrun the wave in a relatively short distance, of the order of a few millimetres to a centimetre – this is called the dephasing limit. While this dephasing limits the maximum energy gain, it has the benefit of generating a monoenergetic electron beam. How does this happen? First, as the radially blown-out plasma electrons rush back toward the axis, a significant number of them are trapped by the longitudinal field of the wake. Second, this self-trapping is severe enough to load the wake with so many electrons that the energy they extract reduces its amplitude, thereby turning off any further trapping – an effect known as beam loading. As the trapped electrons are accelerated their energy initially increases monotonically. However, eventually the electrons in the front dephase and begin to lose energy, while the electrons behind them continue to gain energy (phase-space rotation). This produces a quasi-monoenergetic bunch.
Research groups have now seen such monoenergetic bunches in at least half a dozen laser-wakefield accelerator experiments around the world. Recently the group at Lawrence Berkeley National Laboratory, in collaboration with Oxford University, has used a plasma discharge in a 3.3 cm long capillary tube to produce a hydrogen plasma channel. When the team guided a 40 TW laser pulse through this channel, they produced a monoenergetic beam with an energy up to 1 GeV (Leemans et al. 2006). To go to higher particle energies, laser pulses of even higher power need to be propagated over longer distances in plasma channels. In the next few years we will see if 100 TW class pulses can be guided through plasma channels 10–30 cm long with a plasma density in the range of 1017 cm–3, to produce 10 GeV pulses of high beam quality.
Plasma-wakefield accelerator
There are fewer particle-beam-driven plasma acceleration experiments compared with laser-accelerator experiments. This is because there are fewer suitable beam facilities in the world compared with facilities that can deliver ultra-short laser pulses. The first beam-driven plasma-wakefield experiments were carried out at the Argonne Wakefield Accelerator Facility in the 1980s. Now however, a series of elegant experiments done at SLAC by the UCLA/USC/SLAC collaboration has mapped the physics of electron and positron beam-driven wakes and shown acceleration gradients of 40 GeV/m using electron beams with metre-scale plasmas.

Image credit: MacMillan Publishers Ltd.
In the SLAC experiments only one electron pulse was used to excite the wakefield (Blumenfeld et al. 2007). Since the energy of the drive pulse is nominally 42 GeV, both the electrons and the wake are moving at a velocity close to c, so there is no relative motion between the electrons and the wakefield. Most of the electrons in the drive pulse lose energy in exciting the wake, but some electrons in the back of the same pulse can gain energy from the wakefield as the wakefield changes its sign.
When the 42 GeV SLAC electron beam passed through a column of lithium vapour 85 cm long, the head of the beam created a fully ionized plasma and the remainder of the beam excited a strong wakefield. Figure 2a (p29) shows the energy spectrum of the beam measured after the plasma. The electrons in the bulk of the pulse that lost energy in driving the wake are mostly dispersed out of the field of view of the spectrometer camera and so are not seen in the spectrum. However, electrons in the back of the same pulse are accelerated and reach energies up to 85 GeV. The measured spectrum of the accelerated particles was in good agreement with the spectrum obtained from computer simulations of the experiment, as figure 2b shows. This is a remarkable result when one realises that while it takes the full 3 km length of the SLAC linac to accelerate electrons to 42 GeV, some of these electrons can be made to double their energy in less than a metre.
Where next?
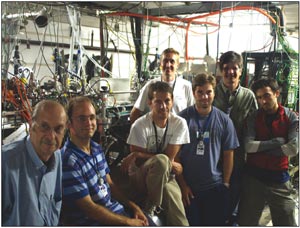
Over the past 25 years, a relatively small number of dedicated researchers have solved many technical problems to reach a point where plasma-based accelerators are producing energy gains of interest to high-energy physics, but there are still many challenges ahead of us. The one that is often brought up is the energy spread and emittance of the accelerated electrons. Laser experiments have already shown self-trapped electron beams with an energy spread of a few per cent. In a beam-driven plasma accelerator a different plasma-electron trapping mechanism, called ionization trapping, could generate a perfectly phased sub-micrometre beam suitable for multi-stage acceleration, with an extremely low emittance and a narrow energy spread. Then there is the issue of the possible degradation of the beam quality because of collisions and, possibly, ion motion. If these are shown to be important effects then, like beam “hosing” and beam “head erosion” they will represent a design constraint on a plasma accelerator (Blumenfeld et al. 2007).
The next key challenge for plasma-based acceleration is to realise high-gradient acceleration of positrons. Positron acceleration is different from electron acceleration in the sense that the focusing forces of positron pulse-generated wakes have nonlinear longitudinal and transverse variation. It may be worthwhile accelerating positrons in linear plasma wakes generated by an electron pulse or by wakefields induced in a hollow channel, but this needs to be demonstrated.
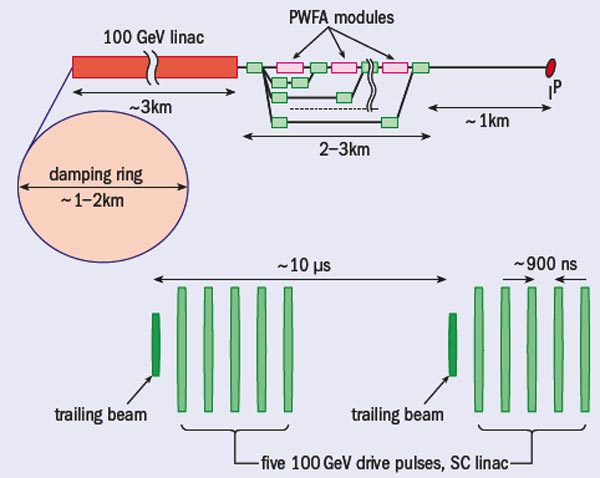
Image credit: Yakimenko and Ischebeck 2006.
Once electron and positron acceleration issues, including energy spread and emittance, have been addressed, the next key development is the “staging” of two plasma-accelerator modules. Again, for high-energy physics applications each module should be designed to add the order of 100 GeV energy to the accelerating beam. Given the microscopic physical size of the accelerating structure (the wavelength is about 100 μm), it is probably wise to minimize the number of plasma acceleration stages. In fact in the proposed energy doubler for the SLAC linac, only a single plasma-wakefield accelerator module was deemed necessary (Lee et al. 2002). In scaling this concept to 1 TeV centre-of-mass energy, one can envision a superconducting linac producing a train of five 100 GeV drive pulses, separated by about 1 μs, but containing three times the charge of the beam pulse that is being accelerated (figure 4). The drive pulses are first separated from one another and subsequently brought back to be colinear with the accelerating beam. Each pulse drives one stage of the plasma-wakefield accelerator from which the accelerating beam gains 100 GeV energy (Yakimenko and Ischebeck 2006). Both electrons and positrons can be accelerated in this manner. Alternatively, one can imagine an e––e– or a γ–γ collider instead of an e––e+ collider, which could greatly reduce the cost of such a machine.
Key challenges
I have described the many fine accomplishments of the advanced acceleration-research community by using the example of plasma-based accelerators. How will this and other concepts for advanced acceleration progress in the next decade? Will they continue to make progress to stay on track for a prototype demonstration of a new accelerator technology in the early 21st century? The answer depends on the availability of one or more suitable experimental facilities to do the next phase of research that I have outlined.
The time is now ripe to invest in appropriate facilities to take this field to the next level
There are several 100 TW class laser facilities in Europe, the US and Asia that should advance the laser-wakefield accelerator to give multi-giga-electron-volt beams. To go beyond this, a high repetition rate, 10 PW class laser facility is needed to demonstrate a 100 GeV prototype of a laser-driven plasma accelerator.
All advanced acceleration schemes will eventually have to face positron acceleration. How and where will experiments on high-gradient positron acceleration be done? The plasma-wakefield accelerator experiments that led to the energy doubling of 42 GeV electrons were carried out at the Final Focus Test Beam (FFTB) at SLAC, which has been recently decommissioned to make way for the Linac Coherent Light Source. SLAC has proposed a “replacement FFTB” beam line called SABER, which will provide experimenters with 30 GeV electron and positron beams. If adequately supported, SABER could become the premier facility not only for plasma-acceleration research but also for other advanced acceleration concepts.
There are about 40 groups worldwide working in plasma-based acceleration with a critical mass of trained scientists and students who are attracted to the field because it offers many chances to make unexpected discoveries. The time is now ripe to invest in appropriate facilities to take this field to the next level. It could be the critical factor that makes the difference to the future of high-energy physics in the 21st century.