What began life as an unwanted energy loss has become a major research industry. Dominique Cornuéjols of the European Synchrotron Radiation Facility in Grenoble looks at the history and accomplishments of synchrotron radiation.

Picture five men around a tabletop ring. From this insignificant 1947 General Electric synchrotron, where the production of light from a particle accelerator was first observed, the newborn beam – “synchrotron radiation” – eventually went on to attract much interest.
However, it began its career on the wrong foot. The emission of light means a loss of energy in accelerator physics, where the main objective is to increase the energy. Who could have foreseen the dramatic evolution that would lead to the construction of large storage rings (erroneously called “synchrotrons”) to which thousands of scientists rush every year for a few days use of this precious light?

Synchrotrons to storage rings
In the beginning, the new light beam was considered a nuisance for particle physics and was too poor to be of interest in other fields. A succession of technological advances went on to propel synchrotron radiation to the forefront of scientific research as an instrument of choice for the study of matter at the atomic and molecular scales.
First there was a switch from synchrotrons to storage rings, with the latter offering a stable beam. Then the rings became larger and their energies rose. Initially, synchrotron radiation was restricted to the visible and near-visible spectral regions, which were already covered by other intense light sources, such as lasers. The spectral range widened progressively, and today it spreads from infrared to gamma rays for the largest rings, with the highest concentration of photons in the X-ray domain.
Access to X-rays has been essential. Their wavelengths are comparable with interatomic distances, so X-ray diffraction by crystalline samples is possible, allowing structures to be analysed at the atomic scale. The other experimental techniques (such as absorption spectroscopy, imaging and scattering) also benefit from the shortening of the wavelength, thus offering a better resolution.
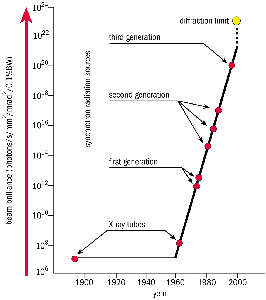
Brilliance
Spectral continuity is certainly a crucial asset of synchrotron radiation. Scientists can select the wavelength best adapted to their study using the beamline monochromator, and they are able to modify their selection during the experiment. Another interesting property, particularly in the X-ray range, is polarization – a synchrotron beam is naturally polarized in the horizontal plane and can also be polarized circularly. Last but not least, the beam is pulsed – a succession of flashes, each lasting about 100 ps, permits the dynamic study of very fast chemical or biological reactions.
However, the most dramatic progress in the past few years has been in the intensity and the collimation of the beam. These two qualities are lumped together in the figure of merit known as brilliance, which compares the optical quality of different sources. Today, the brilliance of the best synchrotron beams is a thousand billion times that of a conventional X-ray source. An excellent brilliance is also accompanied by another quality of the beam – coherence – which used to be laser-specific. Moreover, coherence is obtained by synchrotrons in the X-ray domain that is still difficult for lasers.
How can one explain such an exceptional increase in brilliance? There are two main reasons. The first is the use of special magnetic structures called undulators inside the storage rings. Small permanent magnets are mounted on two jaws with a variable opening. Alternating the magnets’ polarity makes the electrons oscillate inside the structure.
At each oscillation, photons are emitted as a very narrow beam. For instance, if the undulator comprises 100 magnets, the many cones of light superimpose and, by interference, produce a spectrum made of peaks, with the photons accumulated at certain energies. In this way the brilliance of the light beam is considerably increased at the position of the peaks.
By moving the jaws and thus modifying the gap, it is possible to shift the peaks to give the desired energy. Scientists thus benefit from an intense beam for any energy in the accessible range. The most recent machines, known as “third generation” machines, make intensive use of such undulators.
The second explanation for the gain in brilliance is the improvement of the machines, leading to an almost perfect control of the trajectory and shape of the electron beam. The third-generation machines have very low emittances (the product of the size of the beam and its divergence) – the lower the emittance, the higher the brilliance.
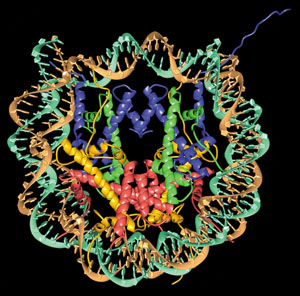
Beamlines
However, the performance of the machine alone is not enough to carry out an experiment using synchrotron light. On the beamline, the beam needs to be treated (essentially monochromatized and focused) to illuminate the sample under optimal conditions.
The third-generation machines have imposed new constraints on the optics. Indeed, due to the intensity of the beam, the heat load is very high and it is necessary to cool the optics (usually with liquid nitrogen). Focusing – always a problem when dealing with X-rays – implies going to the limits of the usual technologies (mirrors) or inventing new devices (lenses) to obtain the microbeams required in a large number of experiments.
The success of sophisticated synchrotron experiments requires highly specialized beamlines. The optics are optimized according to the technique or the research domain. The sample environment has also become a priority: scientists increasingly attempt to reproduce specific experimental conditions. Mechanical constraints, various atmospheres, pressure and temperature are all parameters that have to be controlled in order to carry out experiments, such as those involving catalysis.
Finally, at the end of the beamline comes the detector and the electronics for data acquisition, which, due to the brilliance of the beam, must record a large amount of data in a very short time.
Among the many research domains that make use of synchrotron light, the best example is without any doubt that of protein crystallography. In parallel with genomics, a new science is emerging called proteomics (the study of the proteome – the protein inventory of a living being). While a gene sequence can now be rather easily solved using computer automation, the study of proteins is more complex. In fact, the succession of amino acids in a protein does not directly determine its function: the latter depends on the way in which the protein folds in space, thus forming a three-dimensional structure that evolves during the biological reaction.
Hence, the knowledge of the arrangement of atoms in a biological macromolecule has become one of the major objectives of molecular biology and, naturally, of the pharmaceutical industry too. The structure of small molecules (containing up to a few thousand atoms) can be solved by nuclear magnetic resonance. However, for larger molecules the only available technique is X-ray diffraction (if the protein can be crystallized). Although great progress has been made in crystallization techniques, this operation is often difficult (sometimes impossible) and the crystals obtained are not always of the best quality.
In protein crystallography, synchrotron radiation brings numerous advantages compared with traditional X-rays. In particular it allows the study of minute or weakly diffracting crystals. Also, one can solve very large complexes, associating DNA and RNA with proteins. This is the case for viruses and for the ribosome – the manufacturing plant for proteins. The biggest structure ever solved is that of the blue-tongue virus, which contains some 10 million atoms. Moreover, if one succeeds in crystallizing, for example, a unit made of a viral protein and its antibody, one can learn a lot about the shape of the binding site with high resolution, thus helping the discovery and synthesis of new drugs, perfectly targeted and therefore more efficient.
For a long time, protein crystallography yielded only static images. Thanks to the pulsed structure of synchrotron light and to the brilliance of the new sources, it is now possible to take a sequence of images and identify intermediate states on a nanosecond scale.

Nanostructures
Applied research is constantly probing new material behaviour. For example, significant R&D efforts go into studying the properties of the thin magnetic films used in computer hard disks. A better understanding of new magnetic behaviour, such as magnetoresistance, would considerably increase the storage capacity of these devices. The material normally used for hard-disk recording is a permalloy film, with a magnetoresistance of about 3%. In the sandwiches of iron/chromium/iron or iron/copper/iron, the effect can be as high as 100%.
On a closely related subject, some ultrathin magnetic films of only a few atomic layers show a surprising property – “magnetic perpendicular anisotropy”. The magnetization is not in the plane of the film as might be expected but is instead perpendicular to it, thus giving the possibility of storing many more bits on a given surface.
The dimensions of these structures are on the nanometre scale, so it should no longer be called microelectronics but rather “nanoelectronics”. To study the magnetic properties of these nanostructures, scientists are perfecting new experimental techniques that exploit the qualities of synchrotron radiation. An example is surface diffraction under grazing incidence with an extremely well focused beam, which is used to record only the signal coming from the outermost layers – a technique demanding the highest brilliance.
Another technique perfectly adapted to the study of magnetic materials is magnetic circular dichroism, in which light changes from left to right circular polarization, and vice versa. In some materials the interaction of photons with electrons depends on the direction of the polarization. The resulting difference in absorption reveals the magnetic state of the material and allows the separate measurement of the average moment of each element (iron, copper, cobalt, etc) in the material. No other technique can provide this information.
A large number of synchrotron experiments are carried out in the domain of high pressure. Today we know how to reproduce in the laboratory conditions that are close to those at the centre of the Earth. We need high-pressure cells, where two tapering tips made of diamond squeeze a sample of matter: pressures can reach up to 400 GPa. The samples, which are necessarily very small (a few hundred cubic microns), can additionally be heated using a laser beam. Iron, ice and hydrogen are examples of samples that can be scrutinized through their many crystallographic phases, thus delivering precious information on the behaviour of ultracondensed matter.
Medical applications
Medical research makes use of the new possibilities offered by synchrotron radiation for angiography, tomography and radiotherapy. One of the most notable advances of the last few years is the use of the coherence of the beam in imaging methods: microtomography, when associated with “phase contrast”, allows the exploration of the three-dimensional structure of biological tissues like bones, as well as composite or porous materials (industrial foams, alloys and even snow) with a reinforced contrast and a remarkable resolution.
X-ray microscopy, on the other hand, requires special optics, called zone plates, which advance the resolution limits for imaging to a few tens of nanometres. Compared with other well known microscopy techniques, synchrotron light again offers many advantages, such as the possibility of intensifying contrast by fluorescence due to the presence of certain elements inside the sample.
The range of disciplines in the variety of subjects studied, the continuity between fundamental and applied research, and the constant improvement of experimental devices and techniques to help scientists to go beyond the frontiers of knowledge all promise a bright future for synchrotron radiation.
Virtual visit inside a synchrotron
In real life, only particles can enter the tunnel of the storage ring. X-rays are themselves invisible, and the samples exposed to the beams are often too small to be seen, while the optical devices are protected by vacuum.
A new virtual reconstitution overcomes these visibility barriers and simulates what goes on inside a synchrotron. Conceived as a multimedia encyclopedia, the CD-ROM Synchrotron Light…to Study Matter opens up the world of synchrotrons via hypertext links, interactive illustrations and multiple entries. The numerous two- and three-dimensional animations, as well as the computer-generated images, make the difficult phenomena easy to understand.
This bilingual English/French CD-ROM has been designed for a very wide public. The scientific content has been produced by the ESRF Information Office in collaboration with scientists. The multimedia development has been made by IMediaSoft, a company specializing in multimedia for science, industry and education. The CD-ROM will be distributed worldwide from October by Springer-Verlag.