X-ray free-electron lasers are enabling new classes of experiments
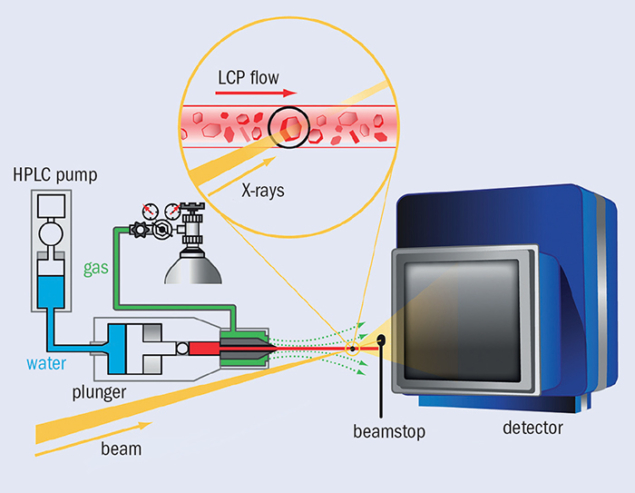
The Linac Coherent Light Source (LCLS) at SLAC produced its first laser-like X-ray pulses in April 2009. The unique and potentially transformative characteristics of the LCLS beam – in particular, the short femtosecond pulse lengths and the large numbers of photons per pulse (see The LCLS XFEL below) – have created whole new fields, especially in the study of biological materials. X-ray diffraction on nanocrystals, for example, reveals 3D structures at atomic resolution, and allows pump-probe analysis of functional changes in the crystallized molecules. New modalities of X-ray solution scattering include wide-angle scattering, which provides detailed pictures from pump-probe experiments, and fluctuational solution scattering, where the X-ray pulse freezes the rotation of the molecules in the beam, resulting in a rich, 2D scattering pattern. Even the determination of the structure of single particles is possible. This article focuses on examples from crystallography and time-resolved solution scattering.
An important example from crystallography concerns the structure of protein molecules. As a reminder, protein molecules, which are encoded in our genes, are linear polymers of the 20 naturally occurring amino-acid monomers. Proteins contain hundreds or thousands of amino acids and carry out most functions within cells or organs. They catalyse chemical reactions; act as motors in a variety of contexts; control the flow of substances into and out of cells; and mediate signalling processes. Knowledge of their atomic structures lies at the heart of mechanistic understanding in modern biology.

Serial femtosecond crystallography (SFX) provides a method of studying the structure of proteins. In SFX, still X-ray photographs are obtained from a stream of nanocrystals, each crystal being illuminated by a single pulse of a few femtoseconds duration. At the LCLS, the 1012 photons per pulse can produce observable diffraction from a protein crystal much less than 1 μm3. Critically, a 10 fs pulse will scatter from a specimen before radiation damage takes place, thereby eliminating such damage as an experimental issue. Figure 1 shows a typical SFX set-up for crystals of membrane proteins. The X-ray beam in yellow illuminates a stream of crystals, shown in the inset, being carried in a thin stream of highly viscous cubic-phase lipid (LCP). The high-pressure system that creates the jet is on the left. The rate of LCP flow is well matched to the 120 Hz arrival rate of the X-ray pulses, so not much material is wasted between shots. In the ideal case, each X-ray pulse scatters from a single crystal in the LCP flow. For soluble proteins, a jet of aqueous buffer replaces the LCP.
AT1R is found at the surface of vascular cells and serves as the principal regulator of blood pressure (figure 3). Although several AT1R blockers (ARBs) have been developed as anti-hypertensive drugs, the structural knowledge of the binding to AT1Rs has been lacking, owing mainly to the difficulties of growing high-quality crystals for structure determination. Using SFX at the LCLS, Vadim Cherezov and colleagues have successfully determined the room-temperature crystal structure of human AT1R in a complex with its selective receptor-blocker ZD7155 at 2.9 Å resolution (Zhang et al. 2015). The structure of the AT1R–ZD7155 complex reveals key features of AT1R and critical interactions for ZD7155 binding. Docking simulations, which predict the binding orientation of clinically used ARBs onto the AT1R structure, further elucidated both the common and distinct binding modes for these anti-hypertensive drugs. The results have provided fundamental insights into the AT1R structure-function relationship and structure-based drug design.

Image credit: Vadim Chezerov.
In solution scattering, an X-ray beam illuminates a volume of solution containing a large number of the particles of interest, creating a diffraction pattern. Because the experiment averages across many rotating molecules, the observed pattern is circularly symmetric and can be encapsulated by a radial intensity curve, I(q), where q = 4πsinθ/λ and 2θ is the scattering angle. The data are therefore essentially one-dimensional (figure 4b). The I(q) curves are quite smooth and can be well described by a modest number of parameters. They have traditionally been analysed to yield a few important physical characteristics of the scattering particle, such as its molecular mass and radius of gyration. Synchrotrons have enabled new classes of solution-scattering experiments, and the advent of XFEL sources is already providing further expansion of the methodology.
Chasing the protein quake
An elegant example of time-resolved wide-angle scattering (WAXS) at the LCLS comes from a group led by Richard Neutze at the University of Gothenberg (Arnlund et al. 2014), which has used multi-photon absorption to trigger an extremely rapid structural perturbation in the photosynthetic reaction centre from Blastochloris viridis, a non-sulphur purple bacterium that produces molecular oxygen valuable to our environment. The group measured the progress of this fluctuation using time-resolved WAXS. Appearing with a time constant of a few picoseconds, the perturbation falls away with a 10 ps time constant and, importantly, precedes the propagation of heat through the protein.

The photosynthetic reaction centre faces unique problems of energy management. The energy of a single photon of green light is approximately equal to the activation energy for the unfolding of the protein molecule. In the photosynthetic complex, photons are absorbed by light-harvesting antennae and then rapidly funnelled to the reaction centre through specialized channels. The hypothesis is that excess energy, which may also be deposited in the protein, is dissipated before damage can be done by a process named “a protein quake”, indicating a nanoscale analogue of the spreading of waves away from the epicentre of an earthquake.
The experiments performed at the coherent X-ray imaging (CXI) station at the LCLS used micro-jet injection of solubilized protein samples. An 800 nm laser pulse of 500 fs duration illuminating the sample was calibrated so that a heating signal could be observed in the difference between the WAXS spectra with and without the laser illumination (figure 5a). The XFEL was operated to produce 40 fs pulses at 120 Hz, and illuminated and dark samples were interleaved, each at 60 Hz. The team calibrated the delay time between the laser and XFEL pulses to within 5 ps, and collected scattering patterns across a series of 41 time delays to a maximum of 100 ps. Figure 5b shows the curves indicating the difference in scattering between activated and dark molecules that were generated at each time point.
The results from this study rely on knowing the equilibrium molecular structure of the complex
The results from this study rely on knowing the equilibrium molecular structure of the complex. Molecular-dynamics (MD) simulations and modelling play a key role in interpreting the data and developing an understanding of the “quake”. A combination of MD simulations of heat deposition and flow in a molecule and spectral decomposition of the time-resolved difference scattering curves provide a strong basis for a detailed understanding of the energy propagation in the system. Because the light pulse was tuned to the frequency of the photosystem’s antennae, cofactors (molecules within the photosynthetic complex) were instantaneously heated to a few thousand kelvin, before decaying with a half-life of about 7 ps through heat flow to the remainder of the protein. Also, principal component analysis revealed oscillations in the range q = 0.2–0.9 nm–1, corresponding to a crystallographic resolution of 31–7 nm, which are signatures of structural changes in the protein. The higher-angle scattering – corresponding to the heat motion – extends to a resolution of a few angstroms, with a time resolution extending to a picosecond. This study illustrates not only the rapid evolution of the technology and experimental prowess of the field, but brings it to bear on a problem that makes clear the biological relevance of extremely rapid dynamics.
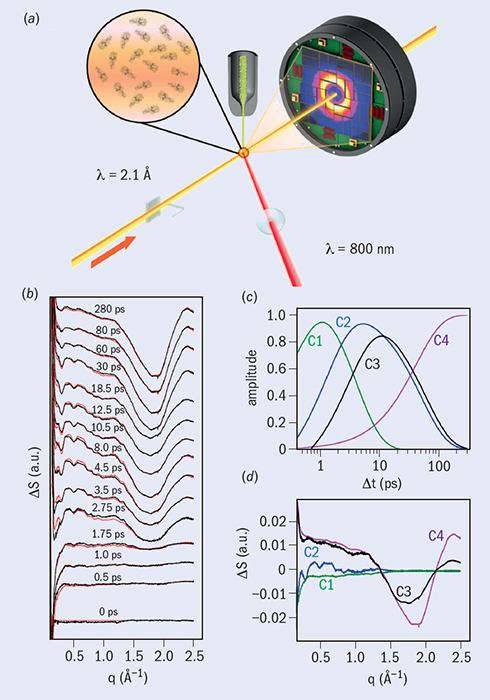
Image credit: Reproduced with permission from Arlund et al.
Effective single-particle imaging (SPI) would eliminate the need for crystallization, and would open new horizons in structure determination. It is an arena in which electron microscopy is making great strides, and where XFELs face great challenges. Simulations have demonstrated the real possibility of recovering structures from many thousands of weak X-ray snapshots of molecules in random orientation. However, it has become clear, as actual experiments are carried out, that there are profound difficulties with collecting high-resolution data – at present the best resolution in 2D snapshot images is about 20 nm. A recent workshop on single-particle imaging at SLAC identified a number of sources of artifacts including complex detector nonlinearities, scattering from apertures, scattering from solvent, and shot-to-shot variation in beam intensity and position. In addition, the current capability to hit a single molecule with a pulse reliably is quite limited. Serious technical progress at XFEL beamlines will be necessary before the promise of SPI at XFELs is realized fully.
Currently, the only operational XFEL facilities are at the SPring-8 Angstrom Compact free-electron LAser (SACLA) at RIKEN in Japan (CERN Courier July/August 2011 p9) and the LCLS in the US, so competition for beamtime is intense. Within the next few years, the worldwide capacity to carry out XFEL experiments will increase dramatically. In 2017, the European XFEL will come on line in Hamburg, providing a pulse rate of 27 kHz compared with the 120 Hz rate at the LCLS. At about the same time, facilities at the Paul Scherrer Institute in Switzerland and at the Pohang Accelerator Laboratory in South Korea will produce first light. In addition, the technologies for performing and analysing experiments are improving rapidly. It seems more than fair to anticipate a rapid growth in crystallography, molecular movies, and other exciting experimental methods.
The LCLS XFEL
Hard X-ray free-electron lasers (XFELs) are derived from the undulator platform commonly used in synchrotron X-ray sources around the world. In the figure, (a) shows the undulator lattice, which comprises a series of alternating pairs of magnetic north and south poles defining a gap through which electron bunches travel. The undulator at the LCLS is 60 m long, compared with about 3 m for a synchrotron device. The bunches experience an alternating force normal to the magnetic field in the gap, transforming their linear path into a low-amplitude cosine trajectory.
In the reference frame of the electron bunch, the radiation that each electron emits has a wavelength equal to the spacing of the undulator magnets (a few centimetres) divided by the square of the relativistic factor γ = E/me2 (see below). Each electron interacts both with the radiation emitted by electrons preceding it in the bunch, and with the magnetic field within the undulator. Initially, the N electrons in the bunch have random phases (see figure, (b)), so that the radiated power is proportional to N.
Image credit: Published with permission from B D Patterson et al. 2010 PCCP 12 647.
As the bunch advances through the undulator, it breaks up into a series of microbunches of electrons separated by the wavelength of the emitted radiation. Without going into detail, this microbunching arises from a Lorenz force on the electron in the direction of propagation, which is generated by the interaction of the undulator field and the (small) component of the electron velocity perpendicular to the direction of propagation. This force tends to push the electrons into a position at the peak of the emitted radiation. All electrons within a single bunch radiate coherently, and the radiation from one microbunch is also coherent with that from the next, being separated by a single wavelength. Therefore, the power in the radiated field is proportional to N2.
The process of microbunching can be viewed as a resonance process, for which the following undulator equation describes the conditions for operation at wavelength λ.
The tables, above, show typical operating conditions for the CXI beamline at the LCLS. The values represent only a small subset of possible operating conditions. Note the small source size, the short pulse duration and the high photons per pulse.
Further reading
D Arnlund et al. 2014 Nature Methods 11 923.
H Zhang et al. 2015 Science in press.
For reviews of the development of XFELs and their applications, see W A Barletta et al. 2010 Nucl. Instrum. and Meth. A 618 69.
A Barty et al. 2013. Annu. Rev. Phys. Chem. 64 415.
J C Spence et al. 2012 Rep. Prog. Phys. 75 102601.
P Thibault and V Elser 2010 Ann. Rev. Cond. Mat. Phys. 1 237.